Author affiliations: Institute of Medical Research and Development, Translational Research Center for Medical Innovation, Foundation for Biomedical Research and Innovation at Kobe, Japan
A neovascularization therapy developed by Translational Research Center for Medical Innovation (TRI) researchers in Japan and based on stem cells is showing great promise for treating chronic critical limb ischemia, offering hope to patients who are out of options.
1. INTRODUCTION
1.1 Pathology, epidemiology and treatment of chronic critical limb ischemia
A circulation disorder caused by the narrowing or occlusion of arteries in the limbs, peripheral arterial disease (PAD) is estimated to affect more than 200 million people globally1, ranging from asymptomatic to severe. The most common underlying disease is arteriosclerosis obliterans (ASO) caused mainly by atherosclerosis; other underlying diseases include thromboangiitis obliterans (also known as Buerger’s disease), vasculitis and autoimmune diseases.
Chronic critical limb ischemia (CLI) is defined as advanced PAD for which sufferers experience pain at rest and have ulcers or gangrene on their lower limbs (Fontaine classification stage III or more severe, or Rutherford classification category 4 or more severe) that persist for two weeks or longer. The end stage of PAD, CLI (also called chronic limb-threatening ischemia recently) generally has a very poor prognosis, being comparable with those of some advanced malignancies. It has a mortality rate of 25%, a survival rate of 30% after major amputation, and a CLI persistence rate of 20% at 1 year. Furthermore, CLI patients have a 5-year survival rate of 40–50%2. The annual incidence of CLI is 500–1,000 per million in Europe and the United States. An estimated 250,000 amputations of lower limbs are performed annually because of CLI1. The global number of patients with PAD has increased by 23.5% between 2000 and 20102, and there is concern that the number of patients may increase rapidly in the future. Amputation of lower limbs not only seriously deteriorates the patient’s quality of life but also causes major social and economic loss.
The currently recommended therapeutic interventions for CLI include pain control, risk factor management, treatment of ulcers or gangrene, and, for suitable patients, revascularization by bypass surgery or endovascular repair3. However, revascularization is unsuitable for approximately 25% to 40% of CLI patients because they lack the vein grafts needed for bypass surgery or have multiple extensive artery lesions or comorbidities1,4,5. The primary goal of CLI treatment is to save the patient’s life and limbs. Given that patients who are not suitable for revascularization or have refractory conditions have a very poor prognosis, it is socially and medically imperative to develop a treatment strategy for such ‘no-option’ CLI patients.
1.2 Neovascularization therapy using CD34+ cells for CLI patients
Basic and clinical research into endothelial progenitor cells (EPCs) took off after 1997, when Asahara and co-workers demonstrated that they are a subset (the CD34+ fraction) of human peripheral blood mononuclear cells (PBMCs)6. Neovascularization therapy involving the transplantation of EPCs has attracted attention as a novel therapy for ischemic diseases. Clinical studies of neovascularization therapy using autologous bone marrow–derived CD34+ cells are being performed globally for diseases such as angina pectoris, acute myocardial infarction, dilated cardiomyopathy, cerebral infarction and CLI.
In 2003, we initiated a world-first phase I/IIa clinical study of lower-limb neovascularization therapy using granulocyte colony stimulating factor (G-CSF)-mobilized autologous CD34+ cells in CLI patients and demonstrated safety and clinical efficacy7. Beginning in 2008, we conducted an investigator-initiated study of a CD34+ cell sorter in compliance with good clinical practice and again demonstrated efficacy and safety8. A multicentre, randomized comparative study (sponsor initiated) was started in December 2017 with the aim of obtaining regulatory approval for CD34+ cells as a regenerative medicine product (Fig. 1). This chapter overviews the background to these studies as well as the prospects for CD34+ cells as a regenerative medicine product.
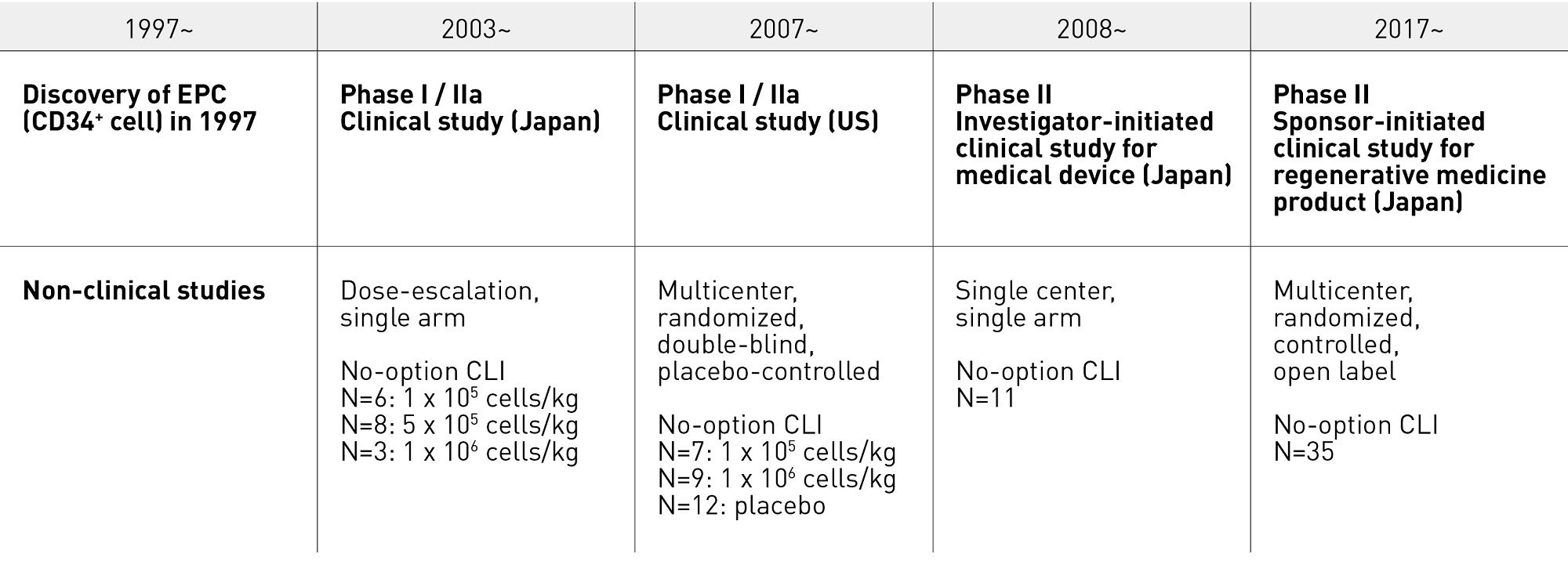
Figure 1. Lower limb neovascularization therapy using G-CSF-mobilized CD34+ cells in CLI patients: path from nonclinical studies to clinical development. CLI, critical limb ischemia; EPC, endothelial progenitor cell; G-CSF, granulocyte colonystimulating factor
2. NONCLINICAL STUDIES
2.1 Characteristics and kinetics of EPCs
Angiogenesis, which involves the proliferation and migration of pre-existing mature vascular endothelial cells, was originally proposed as the vascularization mechanism in adults. However, the identification of EPCs in adults as a subset (CD34+ cells) of PBMCs by Asahara and co-workers in 19976 led to the new concept of vasculogenesis, which differs from angiogenesis in that it involves progenitor cells rather than mature endothelial cells. Neovascularization in adults is now thought to occur through interactions between angiogenesis and vasculogenesis.
EPCs exist within the peripheral blood as mononuclear cells expressing the hematopoietic stem cell surface antigen (CD34) and the early hematopoietic stem cell surface antigen (CD133)9. When isolated and cultured in an endothelial cell growth medium at an appropriate density, these cells differentiate into spindle-shaped adherent cells that express CD34, CD31, vascular endothelial growth factor (VEGF) receptor 2 (also known as kinase insert domain receptor), Tie-2 and E-selectin. Also, their uptake of acetylated low-density lipoprotein has been confirmed6,10.
Basic research using mouse or other animal models of bone-marrow transplantation has shown that EPCs are abundant in the bone marrow in adults. EPCs are forcefully mobilized from the bone marrow to the peripheral blood in conditions such as ischemia, inflammation, wounds, and tumorigenesis, or following the administration of cytokines such as G-CSF and granulocyte and macrophage colony stimulating factor, and hormones such as estrogen. They reach the neovascularization site by circulating blood, where they contribute to the formation of new blood vessels11,12. These findings have been useful for establishing a method for collecting and isolating EPCs for neovascularization therapy (Fig. 2).
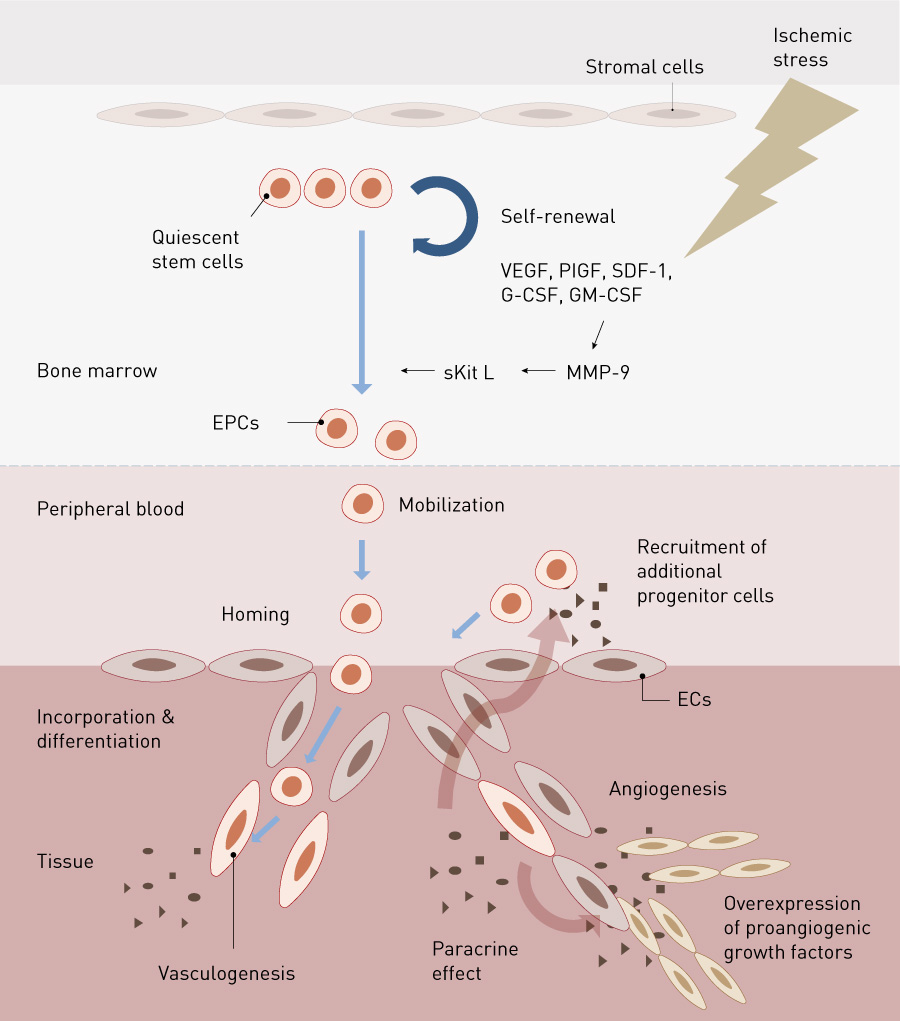
Figure 2. Kinetics of EPCs. EC, endothelial cell; EPC, endothelial progenitor cell; G-CSF, granulocyte colony stimulating factor; GM-CSF, granulocyte macrophage colonystimulating factor; MMP-9, matrix metalloproteinase-9; PlGF, placental growth factor; SDF, stromal cell-derived factor; sKit L, soluble kit ligand; VEGF, vascular endothelial growth factor
Reprinted from Y. Fujita & A. Kawamoto Adv. Drug Deliv. Rev. 120, 25-40 (2017) with permission from Elsevier
2.2 Nonclinical studies on EPC transplantation
Kalka and co-workers obtained EPCs by culturing PBMCs from healthy subjects, and they transplanted them into the nude mouse model of hind-limb ischemia. When EPCs were intravenously injected after two days of hind-limb ischemia, accumulation of transplanted cells at the ischemia site was histologically confirmed, indicating that they contributed to neovascularization in collaboration with mouse-derived vascular endothelial cells. Transplantation significantly improved the capillary density (observed histologically) and blood perfusion in the ischemic limbs (as measured by laser Doppler flowmetry). As a result, the limb salvage rate from necrosis due to severe ischemia was 59% in the EPC transplantation group, compared with only 7–8% in the control group13. Murohara and co-workers reported similar positive results after they collected EPCs from human umbilical cord blood and cultured and transplanted them into nude rats with hind-limb ischemia14. Losordo and co-workers also obtained favourable results after they collected and isolated CD34+ cells from the peripheral blood of healthy subjects who had received a subcutaneous injection of G-CSF and transplanted them into nude rats with hind-limb ischemia (unpublished data).
Nonclinical studies have also shown that EPC transplantation therapy is effective for treating other diseases such as myocardial infarction15,16, cerebral infarction17 and refractory fracture18. These results theoretically support not only EPC transplantation but also transplantation of bone-marrow mononuclear cells (BMMCs, a cell group that includes hematopoietic and mesenchymal cells as well as EPCs). EPCs transplanted into the animal model of ischemia were directly integrated into new blood vessels in the ischemic tissue by vasculogenesis to form the vascular endothelium. The transplanted EPCs were found to produce various cytokines and vascular growth factors involved in angiogenesis, such as VEGF, hepatic growth factor, angiopoietin-1, stromal-cell-derived factor-1 alpha, insulin-like growth factor-1 and endothelial nitric oxide synthase19–21, promoting the proliferation of the existing vascular endothelium and cellular migration (the paracrine effect). Moreover, EPCs have been shown to release not only angiogenesis-related proteins but also RNAs and exosomes containing microRNA, which contribute to the paracrine effect via gene-control mechanisms (Fig. 2)22,23.
While transplantation can be performed using EPCs (CD34+ cells) isolated from BMMCs or PBMCs and purified, many methods for transplanting mononuclear cells (BMMC or PBMC transplantation) without isolating and purifying EPCs have been attempted in nonclinical studies. However, several studies have highlighted the risks associated with transplanting mixed cell groups, including ossification by osteoblasts, chondrification by chondroblasts and fibrosis by fibroblasts. The nonclinical study by Yoon and co-workers also showed that myocardial calcification occurred with high frequency when whole bone marrow cells were transplanted intramyocardially into the rat model of acute myocardial ischemia24. We have also demonstrated that intramyocardial transplant of high-dose PBMCs into the rat model of acute myocardial ischemia led to intramyocardial hemorrhage with infiltration of many inflammatory cells and a reduced improvement in neovascularization and cardiac function. In contrast, transplantation of purified CD34+ cells alone was associated with an absence of adverse reactions, high levels of neovascularization and sustained recovery of cardiac function16. The in vitro EPC colony-forming assay developed by Masuda and co-workers showed that EPC colonies are formed from CD34+ cells at a high frequency, whereas colonies could not be obtained from CD34− mononuclear cells even when using 100 times more cells, demonstrating a marked difference in vascularization potential between the EPC and non-EPC fractions25. These fundamental research and nonclinical studies suggest that transplantation of purified EPCs is superior to BMMC or PBMC transplantation in terms of low invasiveness, therapeutic effect and safety.
3. CLINICAL STUDIES
3.1 Physiological significance of EPCs
Reports have shown that patients with diabetes mellitus and patients with many risk factors for arteriosclerosis have fewer EPCs circulating in their peripheral blood than healthy individuals and that EPCs in these patients have lower proliferative and migratory capacities26,27. Arteriosclerosis patients with fewer EPCs in circulation have many risk factors for poor cardiovascular prognosis and reduced vascular endothelial function28. Thus, EPCs have been shown to be one of the important intrinsic factors that determine the prognosis of patients with arteriosclerosis.
3.2 Phase I/IIa clinical study of neovascularization therapy for CLI patients
Based on the results from the nonclinical studies, we conducted a multicentre, single-blinded, dose-escalation phase I/IIa clinical trial of intramuscular transplantation of G-CSF-mobilized CD34+ cells in CLI patients, which started in 20037. The study enrolled 17 CLI patients (five with ASO, including one on hemodialysis, and 12 with Buerger’s disease). Mononuclear cells mobilized from the bone marrow to the peripheral blood by five days of G-CSF treatment were collected by leukapheresis with high efficiency, and CD34+ cells were further isolated using a magnetic-activated cell sorter (CliniMACS®) (Figs. 3 and 4). The isolated CD34+ cells, which had a mean purity of 92% and a mean cell viability of 87%, were transplanted to the ischemic lower limbs intramuscularly under lumbar spinal anesthesia (Fig. 4). No death or major amputation of lower limbs was reported for any patient in the year following treatment, and all patients maintained independent walking function. Healing of ulcers or necrosis, relief of ischemic pain and improvement over time in physiological measures (for example, toe brachial pressure index (TBI), transcutaneous oxygen partial pressure (TcPO2) were observed, and 88% of patients were free of CLI one year after treatment29. Long-term follow up over the four years following treatment revealed no deaths in the first two years. Four patients died of cardiac diseases after the first two years, but a relationship with the cell therapy was ruled out. No major amputation of lower limbs was reported. The proportion of patients free of CLI remained at over 80% (Fig. 5). Significant improvements were noted in TBI at four years and in TcPO2 at three years compared with pre-treatment data29.
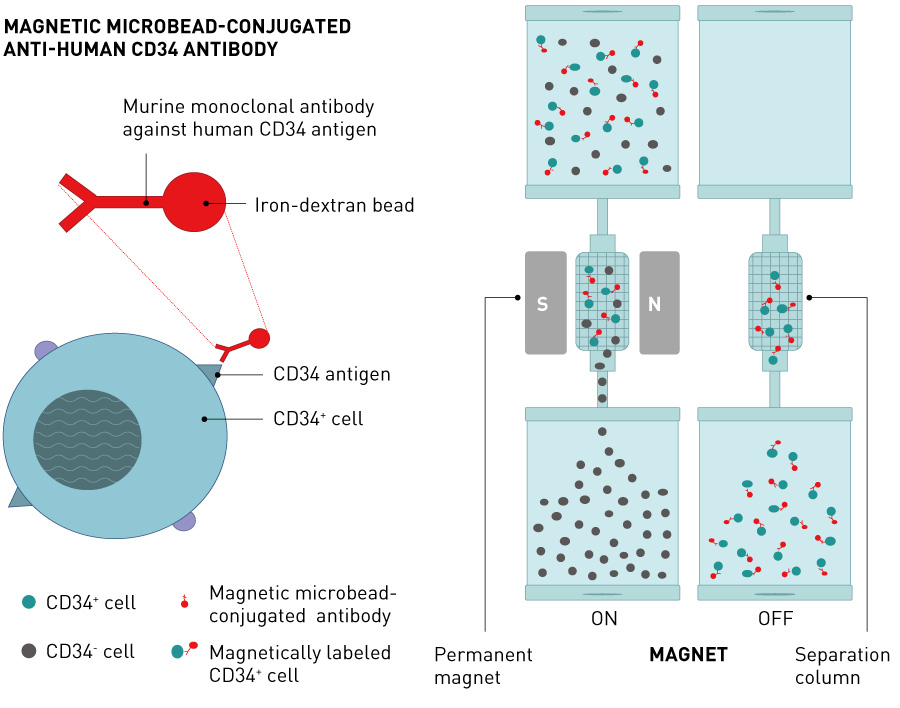
Figure 3. Fundamental principles of magnetic separation of CD34+ cells

Figure 4. Mobilization, harvesting, isolation and transplantation of CD34+ cells in CLI patients EPC, endothelial progenitor cell; G-CSF, granulocyte colony stimulating factor; PMNC, human peripheral blood mononuclear cell
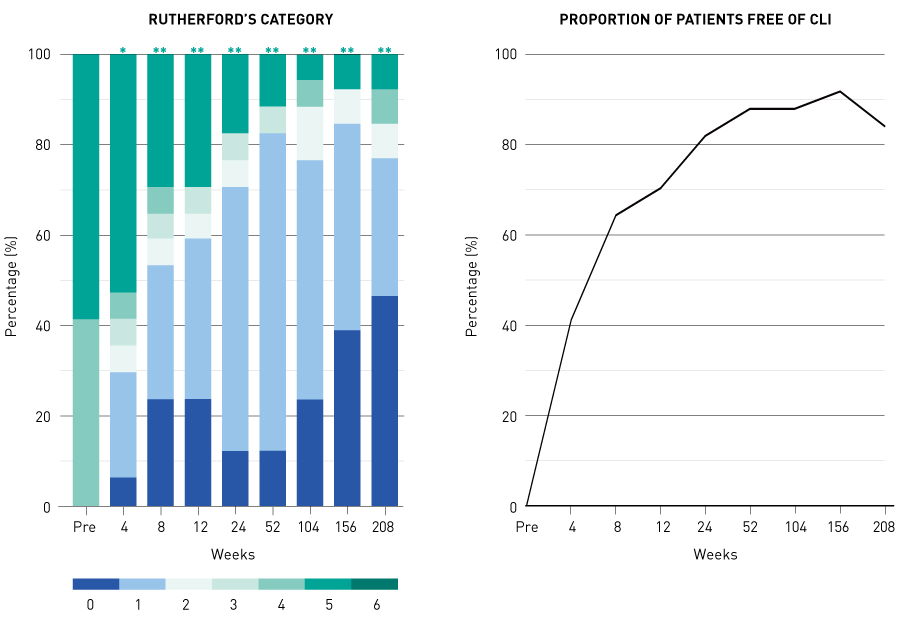
Figure 5. Changes with time in Rutherford’s category and the proportion of CLI patients free of CLI in the four years after transplantation of CD34+ cells: results from the Japanese phase I/IIa clinical study*, P < 0.05; **, P < 0.01 (compared with pre-transplant); CLI, critical limb ischemia.
Reprinted from Ref. 29 with permission from Elsevier
In the United States, Losordo and co-workers initiated a multicentre, randomized, double-blind, placebo-controlled study (study ACT34-CLI) in 2007, using transplantation of G-CSF-mobilized CD34+ cells in 28 patients with CLI caused by ASO30. The incidence of minor and major amputations of lower limbs tended to be lower in the CD34+ cell transplantation group than in the placebo group at 6 months (P = 0.125) and 12 months (P = 0.054) after treatment. No adverse events related to the cell therapy were reported during the one-year follow-up period.
3.3 Investigator-initiated study of CD34+ cell sorter
Following the early clinical studies described above, we sought regulatory approval of the CD34+ cell sorter Isolex®. We began an investigator-initiated study in 2008 in 11 CLI patients (seven with Buerger’s disease and four with ASO who were not on dialysis), in compliance with good clinical practice for medical devices, and completed it in March 2012. This exploratory phase II study was the first investigator-initiated regenerative medicine study in Japan and is still the only Japanese clinical study of lower-limb neovascularization therapy by cell transplantation in which data reliability was assured in accordance with good clinical practice. This investigator-initiated study showed favourable safety and efficacy, as had been previously demonstrated in the phase I/IIa study described above. Measures of signs and symptoms, as well as quality-of-life indices, clinical severity, pain at rest, blood flow improvement indices, and walk test results, were evaluated frequently. Pain at rest improved soon after transplantation of CD34+ cells. Physiological improvement was confirmed, and improvement in Rutherford classification, the clinical severity index, was demonstrated at approximately 6 months (Fig. 6)8.
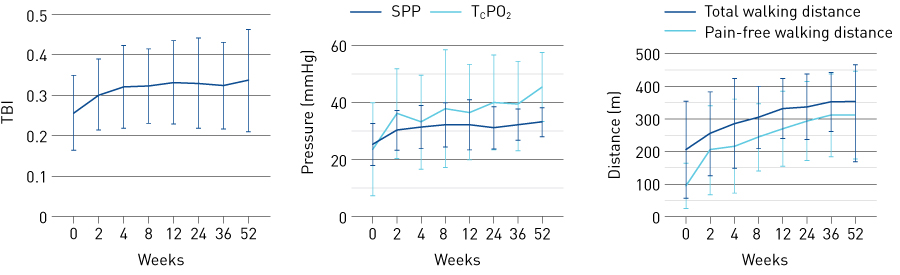
Figure 6. Changes with time in measures of signs and symptoms up to 52 weeks after transplantation of CD34+ cells into CLI patients: results from the exploratory investigator initiated study. SPP, skin perfusion pressure; TBI, toe brachial pressure index; TcPO2, transcutaneous oxygen partial pressure
© 2019 Japanese Circulation Society. Reprinted from Ref. 8
3.4 Sponsor-initiated study aiming for regulatory approval of CD34+ cells as a regenerative-medicine product
Based on the positive results from the phase I/IIa clinical study and the exploratory investigator-initiated study of the medical device, we currently aim to establish a neovascularization therapy of lower limbs using G-CSF-mobilized autologous CD34+ cells as the novel standard of care. When we initiated the exploratory investigator-initiated study in 2008, the company that was intending to file an approval application was trying to develop a CD34+ cell sorter and to file an application for a medical device, but following the completion of our study, the company decided to develop CD34+ cells instead and file an application for a therapeutic cell product.
If a CD34+ cell sorter were developed, it would be installed at each medical institution, and each step — G-CSF administration, apheresis, isolation of CD34+ cells, and cell transplantation — would be completed within the medical institution as a self-contained manufacturing process (Fig. 7). On the other hand, if a therapeutic cell product were developed, G-CSF administration and apheresis would be implemented at each medical institution, and the apheresis product would then be transported to an external cell-processing centre. The CD34+ would be isolated at the cell-processing centre and transported to each medical institution, where transplantation (treatment) would be performed (Fig. 7). Developing a therapeutic cell product has the advantages of easy and wide dissemination (because it is not necessary for every medical institution to install the cell sorter or employ technicians experienced in cell sorting) and a stable supply of high-quality products (because cell products are manufactured by experienced technicians under a more-controlled environment at a cell-processing centre than at general medical institutions). Disadvantages include a high manufacturing cost.
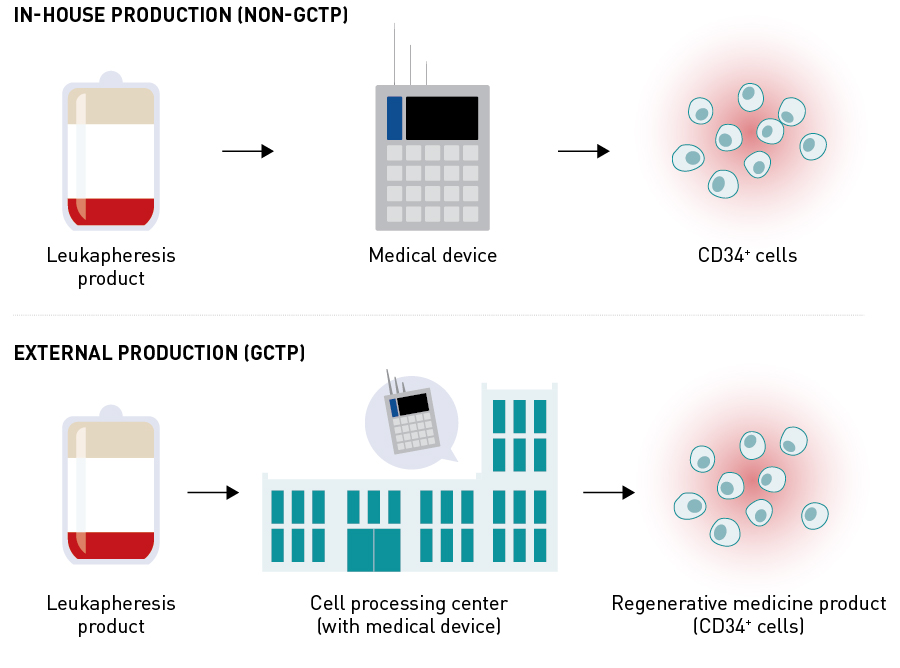
Figure 7. Development of medical device (magnetic cell sorter) and cell product (CD34+ cells) for CD34+ cell therapy during clinical trials. GCTP: Good Gene, Cellular, and Tissue-based Products Manufacturing Practice.
After the company developing the product had changed its plans, the cell-manufacturing process was commissioned to the Foundation for Biomedical Research and Innovation at Kobe. Preparation for a clinical study was initiated in compliance with the structure and facility regulations, cell-manufacturing control, and quality standards based on the Ordinance on Standards of Manufacturing Control and Quality Control for Cellular and Tissue-based Products (Good Gene, Cellular, and Tissue-based Products Manufacturing Practice). In 2013, the Pharmaceutical Affairs Law was amended, and the Law on Securing Quality, Efficacy and Safety of Products including Pharmaceuticals and Medical Devices (Pharmaceutical and Medical Devices Act) was enacted. In addition to pharmaceuticals and medical devices, a new category of regenerative-medicine products was introduced for regulatory review. Following consultation with the Pharmaceuticals and Medical Devices Agency (PMDA) regarding the amendment, we changed our product from the pharmaceutical category to the regenerative-medicine product category. After repeated consultations with the PMDA, the next-phase sponsor-initiated study was designed to be conducted as a multicentre, randomized, comparative study, aiming for regulatory approval of CD34+ cells under the category of regenerative-medicine products. The study was initiated in December 2017, and the first patient was enrolled in the same month. It is currently ongoing. In March 2018, this regenerative-medicine product was designated by the Ministry of Health, Labour and Welfare as an appropriate product for the Sakigake Strategy, which consists of the Sakigake Designation System and the Scheme for Rapid Authorization of Unapproved Drugs. This strategy identifies breakthrough medical devices, pharmaceuticals and regenerative-medicine products that are designed to treat serious diseases and are likely to be developed first in Japan. Such products are then given priority in consultations and reviews to reduce the review time for approval.
4. FUTURE PROSPECTS
The efficacy and safety of this cell therapy have been demonstrated not only for CLI and other ischemic diseases such as refractory angina pectoris31–34, acute myocardial infarction35,36, dilated cardiomyopathy37–42, and cerebral infarction43, but also in refractory fracture44 and liver cirrhosis patients45. The approval of CD34+ cells as a regenerative-medicine product is expected to pave the way for extending application to diseases other than CLI.
References
- Fowkes, F. G., Rudan, D., Rudan, I., Aboyans, V., Denenberg, J. O. et al. Comparison of global estimates of prevalence and risk factors for peripheral artery disease in 2000 and 2010: a systematic review and analysis. Lancet 382, 1329–1340 (2013). | article
- Norgren, L., Hiatt, W. R., Dormandy, J. A., Nehler, M. R., Harris, K. A. et al. Inter-society consensus for the management of peripheral arterial disease (TASC II). J. Vasc. Surg. 45, S5–S67 (2007). | article
- Aboyans, V., Ricco, J.-B., Bartelink, M.-L. E. L., Björck, M., Brodmann, M. et al. 2017 ESC Guidelines on the diagnosis and treatment of peripheral arterial diseases, in collaboration with the European Society for Vascular Surgery (ESVS). Eur. J. Vasc. Endovasc. Surg. 55, 305–368 (2018). | article
- Sprengers, R. W., Moll, F. L. & Verhaar, M. C. Stem cell therapy in PAD. Eur. J. Vasc. Endovasc. Surg. 39, S38–S43 (2010). | article
- Fadini, G. P., Agostini, C. & Avogaro, A. Autologous stem cell therapy for peripheral arterial disease. Atherosclerosis 209, 10–17 (2010). | article
- Asahara, T., Murohara, T., Sullivan, A., Silver, M., van der Zee, R. et al. Isolation of putative progenitor endothelial cells for angiogenesis. Science 275, 964–966 (1997). | article
- Kawamoto, A., Katayama, M., Handa, N., Kinoshita, M., Takano, H. et al. Intramuscular transplantation of G-CSF-mobilized CD34+ cells in patients with critical limb ischemia: A phase I/IIa, multicenter, single-blinded, dose-escalation clinical trial. Stem Cells 27, 2857–2864 (2009). | article
- Fujita, Y., Kinoshita, M., Furukawa, Y., Nagano, T., Hashimoto, H. et al. Phase II clinical trial of CD34+ cell therapy to explore endpoint selection and timing in patients with critical limb ischemia. Circ. J. 78, 490–501 (2014). | article
- Yin, A. H., Miraglia, S., Zanjani, E. D., Almeida-Porada, G., Ogawa, M. et al. AC133, a novel marker for human hematopoietic stem and progenitor cells. Blood 90, 5002–5012 (1997). | article
- Gehling, U. M., Ergün, S., Schumacher, U., Wagener, C., Pantel, K. et al. In vitro differentiation of endothelial cells from AC133-positive progenitor cells. Blood 95, 3106–3112 (2000). | article
- Takahashi, T., Kalka, C., Masuda, H., Chen, D., Silver, M. et al. Ischemia- and cytokine-induced mobilization of bone marrow-derived endothelial progenitor cells for neovascularization. Nat. Med. 5, 434–438 (1999).| article
- Asahara, T., Masuda, H., Takahashi, T., Kalka, C., Pastore, C. et al. Bone marrow origin of endothelial progenitor cells responsible for postnatal vasculogenesis in physiological and pathological neovascularization. Circ. Res. 85, 221–228 (1999). | article
- Kalka, C., Masuda, H., Takahashi, T., Kalka-Moll, W. M., Silver, M. et al. Transplantation of ex vivo expanded endothelial progenitor cells for therapeutic neovascularization. Proc. Natl. Acad. Sci. USA 97, 3422–3427 (2000). | article
- Murohara, T., Ikeda, H., Duan, J., Shintani, S., Sasaki, K. et al. Transplanted cord blood-derived endothelial precursor cells augment postnatal neovascularization. J. Clin. Invest. 105, 1527–1536 (2000). | article
- Kawamoto, A., Gwon, H. C., Iwaguro, H., Yamaguchi, J. I., Uchida, S. et al. Therapeutic potential of ex vivo expanded endothelial progenitor cells for myocardial ischemia. Circ. 103, 634–637 (2001).| article
- Kawamoto, A., Iwasaki, H., Kusano, K., Murayama, T., Oyamada, A. et al. CD34-positive cells exhibit increased potency and safety for therapeutic neovascularization after myocardial infarction compared with total mononuclear cells. Circ. 114, 2163–2169 (2006). | article
- Taguchi, A., Soma, T., Tanaka, H., Kanda, T., Nishimura, H. et al. Administration of CD34+ cells after stroke enhances neurogenesis via angiogenesis in a mouse model. J. Clin. Invest. 114, 330–338 (2004). | article
- Fukui, T., Mifune, Y., Matsumoto, T., Shoji, T., Kawakami, Y. et al. Superior potential of CD34-positive cells compared to total mononuclear cells for healing of nonunion following bone fracture. Cell Trans. 24, 1379–1393 (2015). | article
- Li, M., Nishimura, H., Iwakura, A., Wecker, A., Eaton, E. et al. Endothelial progenitor cells are rapidly recruited to myocardium and mediate protective effect of ischemic preconditioning via “imported” nitric oxide synthase activity. Circ. 111, 1114–1120 (2005). | article
- Jujo, K., li, M. & Losordo, D. W. Endothelial progenitor cells in neovascularization of infarcted myocardium. J. Mol. Cell Cardiol. 45, 530–544 (2008). | article
- Miyamoto, Y., Suyama, T., Yashita, T., Akimaru, H. & Kurata, H. Bone marrow subpopulations contain distinct types of endothelial progenitor cells and angiogenic cytokine-producing cells. J. Mol. Cell Cardiol. 43, 627–635 (2007). | article
- Valadi, H., Ekström, K., Bossios, A., Sjöstrand, M., Lee, J. J. et al. Exosome-mediated transfer of mRNAs and microRNAs is a novel mechanism of genetic exchange between cells. Nat. Cell Biol. 9, 654–659 (2007). | article
- Sahoo, S., Klychko, E., Thorne, T., Misener, S., Schultz, K. M. et al. Exosomes from human CD34+ stem cells mediate their proangiogenic paracrine activity. Circ. Res. 109, 724–728 (2011). | article
- Yoon, Y.-S., Park, J.-S., Tkebuchava, T., Luedeman, C. & Losordo, D. W. Unexpected severe calcification after transplantation of bone marrow cells in acute myocardial infarction. Circ. 109, 3154–3157 (2004). | article
- Masuda, H., Alev, C., Akimaru, H., Ito, R., Shizuno, T. et al. Methodological development of a clonogenic assay to determine endothelial progenitor cell potential. Circ. Res. 109, 20–37 (2011). | article
- Vasa, M., Fichtlscherer, S., Aicher, A., Adler, K., Urbich, C. et al. Number and migratory activity of circulating endothelial progenitor cells inversely correlate with risk factors for coronary artery disease. Circ. Res. 89, E1–E7 (2001). | article
- Tepper, O. M., Galiano, R. D., Capla, J. M., Kalka, C., Gagne, P. J. et al. Human endothelial progenitor cells from type II diabetics exhibit impaired proliferation, adhesion, and incorporation into vascular structures. Circ. 106, 2781–2786 (2002). | article
- Hill, J. M., Zalos, G., Halcox, J. P., Schenke, W. H., Waclawiw, M. A. et al. Circulating endothelial progenitor cells, vascular function, and cardiovascular risk. New Engl. J. Med. 348, 593–600 (2003). | article
- Kinoshita, M., Fujita, Y., Katayama, M., Baba, R., Shibakawa, M. et al. Long-term clinical outcome after intramuscular transplantation of granulocyte colony stimulating factor-mobilized CD34 positive cells in patients with critical limb ischemia. Atherosclerosis 224, 440–445 (2012). | article
- Losordo, D. W., Kibbe, M. R., Mendelsohn, F., Marston, W., Driver, V. R. et al. A randomized, controlled pilot study of autologous CD34+ cell therapy for critical limb ischemia. Circ. Cardiovasc. Interv. 5, 821–830 (2012). | article
- Povsic, T. J., Henry, T. D., Traverse, J. H., Fortuin, F. D., Schaer, G. L. et al. The RENEW Trial: Efficacy and safety of intramyocardial autologous CD34+ cell administration in patients with refractory angina. JACC Cardiovasc. Interv. 9, 1576–1585 (2016). | article
- Losordo, D. W., Schatz, R. A., White, C. J., Udelson, J. E., Veereshwarayya, V. et al. Intramyocardial transplantation of autologous CD34+ stem cells for intractable angina: a phase I/IIa double-blind, randomized controlled trial. Circ. 115, 3165–3172 (2007). | article
- Losordo, D. W., Henry, T. D., Davidson, C., Sup Lee, J., Costa, M. A. et al. Intramyocardial, autologous CD34+ cell therapy for refractory angina. Circ. Res. 109, 428–436 (2011). | article
- Henry, T. D., Losordo, D. W., Traverse, J. H., Schatz, R. A., Jolicoeur, E. M. et al. Autologous CD34+ cell therapy improves exercise capacity, angina frequency and reduces mortality in no-option refractory angina: a patient-level pooled analysis of randomized double-blinded trials. Eur. Heart J. 39, 2208–2216 (2018). | article
- Quyyumi, A. A., Waller, E. K., Murrow, J., Esteves, F., Galt, J. et al. CD34+ cell infusion after ST elevation myocardial infarction is associated with improved perfusion and is dose dependent. Am. Heart J. 161, 98–105 (2011). | article
- Quyyumi, A. A., Vasquez, A., Kereiakes, D. J., Klapholz, M., Schaer, G. L. et al. PreSERVE-AMI: A randomized, double-blind, placebo-controlled clinical trial of intracoronary administration of autologous CD34+ cells in patients with left ventricular dysfunction post STEMI. Circ. Res. 120, 324–331 (2017). | article
- Vrtovec, B., Poglajen, G., Sever, M., Lezaic, L., Domanovic, D. et al. Effects of intracoronary stem cell transplantation in patients with dilated cardiomyopathy. J. Card. Fail. 17, 272–281 (2011). | article
- Vrtovec, B., Poglajen, G., Lezaic, L., Sever, M., Socan, A. et al. Comparison of transendocardial and intracoronary CD34+ cell transplantation in patients with nonischemic dilated cardiomyopathy. Circ. 128, S42–S49 (2013). | article
- Vrtovec, B., Poglajen, G., Lezaic, L., Sever, M., Domanovic, D. et al. Effects of intracoronary CD34+ stem cell transplantation in nonischemic dilated cardiomyopathy patients: 5-year follow-up. Circ. Res. 112, 165–173 (2013). | article
- Lezaic, L., Socan, A., Poglajen, G., Peitl, P. K., Sever, M. et al. Intracoronary transplantation of CD34+ cells is associated with improved myocardial perfusion in patients with nonischemic dilated cardiomyopathy. J. Card. Fail. 21, 145–152 (2015). | article
- Frljak, S., Jaklic, M., Zemljic, G., Cerar, A., Poglajen, G. et al. CD34+ cell transplantation improves right ventricular function in patients with nonischemic dilated cardiomyopathy. Stem Cells Transl. Med. 7, 168–172 (2018). | article
- Bervar, M., Kozelj, M., Poglajen, G., Sever, M., Zemljic, G. et al. Effects of transendocardial CD34+ cell transplantation on diastolic parameters in patients with nonischemic dilated cardiomyopathy. Stem Cells Transl. Med. 6, 1515–1521 (2017). | article
- Banerjee, S., Bentley, P., Hamady, M., Marley, S., Davis, J. et al. Intra-arterial immunoselected CD34+ stem cells for acute ischemic stroke. Stem Cells Transl. Med. 3, 1322–1330 (2014). | article
- Kuroda, R., Matsumoto, T., Niikura, T., Kawakami, Y., Fukui, T. et al. Local transplantation of granulocyte colony stimulating factor-mobilized CD34+ cells for patients with femoral and tibial nonunion: pilot clinical trial. Stem Cells Transl. Med. 3, 128–134 (2014). | article
- Nakamura, T., Torimura, T., Iwamoto, H., Kurogi, J., Inoue, H. et al. CD34+ cell therapy is safe and effective in slowing the decline of hepatic reserve function in patients with decompensated liver cirrhosis. J. Gastroenterol. Hepatol. 29, 1830–1838 (2014). | article