Author Affiliations: *Department of Circulatory and Respiratory Advanced Medicine, Gifu University Graduate School of Medicine, Gifu, Japan; †Heart Failure Center, Gifu Municipal Hospital, Gifu, Japan; ‡Department of Cardiology, Gifu University Graduate School of Medicine, Gifu, Japan; §Department of Stem Cell Biology and Histology, Tohoku University Graduate School of Medicine, Sendai, Japan
Multilineage-differentiating stress enduring (Muse) cells are promising for treating acute myocardial infarction. Specifically, animal studies conducted by researchers associated with the Translational Research Center for Medical Innovation (TRI) in Japan have shown that they improve cardiac function and attenuate left ventricular remodelling after acute myocardial infarction.
1. ACUTE MYOCARDIAL INFARCTION: CURRENT TREATMENT AND THERAPEUTIC CHALLENGES
Acute myocardial infarction (AMI) is caused by a sudden occlusion of the coronary artery. The occlusion blocks blood flow, which delivers oxygen and nutrients to the myocardial tissue, and hence causes necrosis of the tissue. AMI leads to heart failure because of reduced cardiac function and left ventricular (LV) remodelling and is associated with high mortality1.
After complete occlusion of the coronary artery, necrosis commences in the ventricular wall in the region that has lost the blood supply by the occluded coronary artery. Necrosis then gradually extends from the endocardium towards the epicardium. Because of this progression of necrosis, which is termed a wavefront phenomenon2, early reperfusion after myocardial ischemia can confine myocardial necrosis to the subendocardial region, resulting in a smaller infarct size and less impairment of cardiac function. However, failure to perform early reperfusion results in a transmural infarct that extends from the endocardium to the epicardium. If the coronary artery involved is a large proximal blood vessel, massive myocardial infarction occurs and seriously impairs cardiac function. In the subacute and chronic phases, the infarcted LV wall undergoes remodelling, which thins the wall and increases the LV chamber diameter. This remodelling can lead to heart failure. Consequently, one of the major problems in treating AMI is that patients saved in the acute phase (about 1 week after onset) still have a risk of heart failure in the chronic phase and may have a poor prognosis.
The current first-line and most effective therapy for AMI is reperfusion of the occluded coronary artery, implemented as early as possible after AMI onset to save residual viable cardiomyocytes and minimize damage to the myocardium. The therapy involves diagnosing as soon as possible after AMI and transporting the patient to a catheterization laboratory, where the occluded coronary artery is revascularized by percutaneous coronary intervention (PCI) to restore blood flow to myocardial tissue. Patients who received reperfusion within 60 minutes from AMI onset had a good prognosis, whereas prognosis was significantly poorer if reperfusion was delayed for longer than 120 minutes3. Reperfusion by PCI within 90 minutes of onset is considered the gold standard, but AMI patients commonly fail to receive therapy within 90 minutes, if they receive it at all4. Reperfusion may also fail. Therefore, AMI treatment with reperfusion by PCI alone is not enough to improve prognosis.
The process of LV remodelling during the transition from the subacute to the chronic phase is complex and closely related to the healing process, including hypertrophy of residual cardiomyocytes and reconstruction of the LV by non-cardiomyocytes such as stromal cells5,6. The extent to which cardiac function recovers after AMI depends on the effect of remodelling on the healing process of the AMI lesion. If pluripotent stem cells that can differentiate into cardiomyocytes could be efficiently engrafted into the infarcted area and replace dead cardiomyocytes during the healing process, cardiac function impairment and LV remodelling, as well as heart failure in the chronic phase, could be prevented and the patient’s prognosis improved. Autologous stem cells derived from the bone marrow (BM) have been used, and various regenerative effects have been reported7,8. Clinical application of autologous cells should be straightforward since there are no ethical concerns with their use. Many clinical studies of tissue repair and regeneration therapy after AMI have been conducted worldwide using BM nucleated cells and BM-derived mesenchymal stem cells (MSCs)7,8. In placebo-controlled studies, the therapy showed no or minimal improvement in cardiac function in the chronic phase7,8. These limitations of postinfarct regenerative therapy using BM nucleated cells or BM-derived MSCs as a cell source have prompted a search for an alternative cell source that readily differentiates into cardiomyocytes and has a potent repair and regenerative ability.
In 2010, Dezawa et al. discovered multilineage-differentiating stress enduring (Muse) cells9. Muse cells are selected as cells positive for stage-specific embryonic antigen-3 (SSEA-3), a cell surface marker for pluripotent stem cells9–12. Muse cells also express pluripotency markers such as Sox2, Oct3/4 and Nanog, and they can differentiate into cells of all three germ layers (ectodermal, mesodermal and endodermal lineage cells) from a single cell9. Moreover, Muse cells reside naturally in the human body and are located in the BM9, skin10, adipose tissue12,13, connective tissue of various organs14 and peripheral blood15,16. Because Muse cells are pluripotent stem cells and the therapy does not require gene transfer, they should be safe and clinical application should be straightforward. Reports have shown that about 1 million Muse cells can be collected from approximately 30 mL of fresh human BM aspirates cultured for 3 days14,17. This ease of culture suggests that therapy using Muse cells are considered feasible for clinical application.
2. MUSE CELL NUMBERS IN THE BLOOD OF ACUTE-PHASE AMI PATIENTS AFFECT CARDIAC FUNCTION AND LV REMODELLING IN THE CHRONIC PHASE
The existence of Muse cells in the peripheral blood has previously been documented for ischemic stroke patients15, but the kinetics of Muse cells in the peripheral blood of AMI patients during the acute phase had not been characterized. We thus investigated changes in the number of Muse cells in the blood during the acute phase in 79 AMI patients admitted to Gifu University Hospital (AMI group). We also investigated the effect of the change in Muse cell numbers on cardiac function and LV remodelling 6 months after the onset of AMI. The results were compared with two further groups: a coronary artery disease (CAD) group (n = 44) consisting of patients with ≥75% coronary artery stenosis on coronary angiography and a control group (n = 64) consisting of patients with <75% coronary artery stenosis. Muse cell numbers (cells/100 μL) in the peripheral blood were determined as SSEA3+/CD105+ double-positive cells by fluorescence-activated cell sorting16. Muse cell numbers in the blood were measured on the day of admission (day 0) and at 1, 7, 14 and 21 days after AMI.
We obtained the following results16. No significant difference was detected in Muse cell numbers between the AMI group and the control group on the day of admission. Muse cell numbers in the AMI group peaked 24 hours after AMI onset and were significantly higher than on the day of admission. This significant increase was maintained at 7 days. After 14 days, the Muse cell numbers had decreased significantly, and by 21 days they had returned to baseline (Fig. 1A). Muse cell numbers in the blood in the CAD and control groups were not significantly different (Fig. 1B).
![Figure 1. A, Changes in Muse cell numbers with time in the peripheral blood of AMI patients. B, Muse cell numbers in the peripheral blood of AMI patients, CAD patients and subjects with normal coronary arteries (control). C, Relationship between the increase in the number of Muse cells (ΔMuse) in the acute phase and cardiac function improvement in the chronic phase measured as the change in ejection fraction (ΔEF). D, Relationship between ΔMuse in the acute phase and ΔLVDd in the chronic phase. * p < 0.05; ** p < 0.01; LVDd, left ventricular end-diastolic dimension. [Reproduced from Ref. 16]](../../cms/figure/index/5d8d933876c90031655acc8d.jpg)
Figure 1. A, Changes in Muse cell numbers with time in the peripheral blood of AMI patients. B, Muse cell numbers in the peripheral blood of AMI patients, CAD patients and subjects with normal coronary arteries (control). C, Relationship between the increase in the number of Muse cells (ΔMuse) in the acute phase and cardiac function improvement in the chronic phase measured as the change in ejection fraction (ΔEF). D, Relationship between ΔMuse in the acute phase and ΔLVDd in the chronic phase. * p < 0.05; ** p < 0.01; LVDd, left ventricular end-diastolic dimension. [Reproduced from Ref. 16]
From Ref. 16 © 2019 Japanese Circulation Society
These results demonstrate that Muse cell numbers in the peripheral blood do not increase in CAD patients with significant coronary artery stenosis, but are mobilized into blood only when AMI occurs, causing necrosis of myocardial tissue. MAX Muse was defined as the maximum number of Muse cells measured within 21 days of admission due to AMI (up to 21 days), and ΔMuse was defined as the difference in Muse cell numbers between MAX Muse and the minimum number. Both the peak creatine kinase (PCK) level after AMI onset and ΣCK, the sum of creatine kinase levels, are considered measures of myocardial infarct size18. A high ΔMuse in patients with a high PCK or ΣCK was associated with a large infarct size; that is, the more damaged the myocardium was, the more Muse cells were mobilized. We also measured plasma sphingosine-1-phosphate (S1P) levels by liquid chromatography–tandem mass spectrometry. Plasma S1P levels in the AMI group were significantly higher than in the control group, whereas plasma S1P levels in the CAD and control groups were not significantly different. Plasma S1P levels and MAX Muse showed a significant positive correlation (r = 0.330)16. These results suggest that S1P might be involved in mobilizing Muse cells in the blood16.
We then investigated the effect of the number of Muse cells mobilized in the acute phase on cardiac function and LV remodelling in the chronic phase (at 6 months). The results were as follows. ΔMuse (maximum – minimum Muse cell number) was significantly higher in patients with improved cardiac function, as measured by ejection fraction (EF), in the chronic phase (at 6 months) than in patients without an improvement in EF (Fig. 1C); ΔMuse was also significantly higher in patients with decreased LV end-diastolic dimension (LVDd) (that is, attenuated LV remodelling) than in patients with increased LVDd (that is, accelerated LV remodelling) in the chronic phase (Fig. 1D). These results demonstrate that cardiac function is improved and LV remodelling is attenuated in the chronic phase if large numbers of Muse cells are mobilized into the peripheral blood during the acute phase of AMI16. Administering exogenous Muse cells in the acute phase is thus expected to improve cardiac function and attenuate LV remodelling in the chronic phase.
3. TISSUE REPAIR/REGENERATION THERAPY FOR INFARCTED MYOCARDIUM USING MUSE CELLS
3.1 Effect on infarct size, cardiac function, and LV remodelling
We conducted an in vitro study to investigate the differentiation of Muse cells into cardiomyocytes by culturing rabbit BM-derived Muse cells and human BM-derived Muse cells. Muse cells differentiated into cardiomyocyte-like cells that expressed cardiac troponin I and sarcomeric α-actinin19. To develop a tissue repair and regeneration therapy for human MI using Muse cells and to demonstrate the efficacy and safety of the therapy in vivo, we developed a rabbit AMI model by occluding the coronary artery of Japanese white rabbits for 30 minutes and reperfusing it (ischemia reperfusion model)19.
The current first-line therapy for AMI is revascularization of the occluded coronary artery by PCI using a cardiac catheter. Therapy using autograft Muse cells would be worth developing if it were found to be more effective in improving cardiac function and attenuating LV remodelling than stem-cell therapies primarily involving autograft MSCs, which have been used in clinical studies to date.
The effects of 3 × 105 autograft Muse cells intravenously infused into rabbit 24 hours after AMI onset (the Muse group) were compared with an equal number of autograft MSCs (the MSC group). As MSCs include several percent of Muse cells, the effects of MSCs that do not include Muse cells (the non-Muse group) were also studied. The parameters studied were the myocardial infarct size and those that indicate LV function: LV ejection fraction (EF%), LV fractional shortening (FS%), LV systolic function (+dP/dt) and LV diastolic function (−dP/dt); and those that indicate LV remodelling: LVDs and LVDd. These parameters were compared 2 weeks and 2 months after AMI in the Muse, non-Muse and MSC groups, as well as in a control group, which was injected with vehicle only. The results showed that the MSCs moderately reduced infarct size at both 2 weeks and 2 months compared with the control group, but that there was a marked reduction in infarct size in the Muse group, approximately two times greater than in the MSC group (Fig. 2A and 2B). The attenuating effect on LV remodelling and the effect on cardiac function improvement were also significantly greater in the Muse group than in the MSC group at 2 weeks and 2 months. The greater improvement achieved using Muse cells compared with conventional stem cells (MSCs) indicates that development of tissue repair/regeneration therapy using Muse cells for AMI would be clinically very significant.
![Figure 2. A, Effects of autograft Muse cells, non-Muse cells and MSCs on infarct size (2 weeks after AMI). B, Effects of autograft Muse cells, non-Muse cells, and MSCs on infarct size (2 months after AMI). * p < 0.05; ** p < 0.01; *** p < 0.001; AMI, acute myocardial infarction; MSC, mesenchymal stem cell. [Reproduced from Ref. 19]](../../cms/figure/index/5d8d934c76c90031655acc91.jpg)
Figure 2. A, Effects of autograft Muse cells, non-Muse cells and MSCs on infarct size (2 weeks after AMI). B, Effects of autograft Muse cells, non-Muse cells, and MSCs on infarct size (2 months after AMI). * p < 0.05; ** p < 0.01; *** p < 0.001; AMI, acute myocardial infarction; MSC, mesenchymal stem cell. [Reproduced from Ref. 19]
From Ref. 19 © 2019 American Heart Association, Inc.
As we anticipated, AMI treatment using Muse cells could potentially be more effective than conventional therapies. However, some problems arise when autograft Muse cells are used in clinical practice. Collecting BM aspirates from patients immediately after AMI onset in the acute phase is an invasive procedure. At least 7 days is required to isolate Muse cells from BM aspirates and culture a sufficient number of Muse cells for tissue repair and regeneration. It is thus more realistic in clinical practice to use allograft Muse cells, which can be administered within the optimal time window, instead of autograft Muse cells. When 3 × 105 allograft Muse cells derived from rabbit BM cells were intravenously infused into rabbits 24 hours after AMI onset, the reduction in infarct size at 2 weeks was comparable to that seen with autograft Muse cells19. The effect of allograft Muse cells persisted for a long time - the effects of allograft Muse cells in reducing infarct size, improving LV function and attenuating LV remodelling were sustained for 6 months after AMI19.
3.2 Mechanism of engraftment of Muse cells into the infarct region
One remarkable characteristic of Muse cells intravenously injected after AMI was preferential engraftment into the infarct area. Engraftment in the MI site was investigated using Muse and non-Muse cells labelled with the luminescent Nano-lantern protein. Muse cells were engrafted into the infarct region but non-Muse cells were not (Fig. 3A). Co-injection of Muse cells with JTE-013, an antagonist selective for sphingosine-1-phosphate receptor 2 (S1PR2), significantly blocked the engraftment of Muse cells onto the infarct region (Fig. 3B). Histological analyses of Muse cell engraftment 3 days after AMI also showed that Muse cells were engrafted into the infarct area, and that this engraftment was substantially inhibited by co-injection with JTE-013 (Fig. 3C). The engraftment rate of Muse cells was 14.5% at 3 days19. Given that the engraftment rates usually reported for MSCs are 0–3%20,21, the rate for Muse cells is high. The S1P levels were significantly higher in the infarct area and the infarct border area than in the corresponding areas in normal myocardial tissue (Fig. 3D). This result is due to the preferential engraftment of intravenously injected Muse cells into the border area and the infarct area. An in vitro assay using a Boyden chamber to quantify Muse cell migration showed that the number of migrated Muse cells increased significantly and in a dose-dependent manner in response to the S1PR2 agonist SID46371153. A marked level of migration of Muse cells towards affected cardiac tissue was observed, but this migration was blocked in a dose-dependent manner by JTE-013. S1PR2 is expressed on the surface of Muse cells. Silencing S1PR2 on Muse cells using siRNA significantly inhibited migration of Muse cells towards the S1PR2 agonist SID46371153 or AMI cardiac tissue19. These in vivo and in vitro data indicate that the S1P–S1PR2 axis is involved in the mechanisms of migration and engraftment of Muse cells into AMI cardiac tissue.
![Figure 3. A, Nano-lantern-labelled Muse cells accumulated in the infarct area, but non-Muse cells did not (2 weeks after AMI). B, Nano-lantern-labelled Muse cells detected in the infarct area did not accumulate when co-injected with JTE-013, an antagonist selective for S1PR2 (2 weeks after AMI). C, Numbers of Muse cells engrafted in the infarct area with and without JTE-013 estimated from immunohistochemical analysis 3 days after AMI. D, S1P levels in the infarct area and the border area of infarcted and normal myocardial tissue. S1P, sphingosine-1-phosphate; S1PR2, sphingosine-1-phosphate receptor 2 * p < 0.05; ** p < 0.01; *** p < 0.001. [Reproduced from Ref. 19]](../../cms/figure/index/5d8d935776c90029d46053ac.jpg)
Figure 3. A, Nano-lantern-labelled Muse cells accumulated in the infarct area, but non-Muse cells did not (2 weeks after AMI). B, Nano-lantern-labelled Muse cells detected in the infarct area did not accumulate when co-injected with JTE-013, an antagonist selective for S1PR2 (2 weeks after AMI). C, Numbers of Muse cells engrafted in the infarct area with and without JTE-013 estimated from immunohistochemical analysis 3 days after AMI. D, S1P levels in the infarct area and the border area of infarcted and normal myocardial tissue. S1P, sphingosine-1-phosphate; S1PR2, sphingosine-1-phosphate receptor 2 * p < 0.05; ** p < 0.01; *** p < 0.001. [Reproduced from Ref. 19]
From Ref. 19 © 2019 American Heart Association, Inc.
3.3 Differentiation of Muse cells into cardiomyocytes
Thin sections of affected cardiac tissue were stained 2 weeks after AMI to investigate the expression of cardiac markers atrial natriuretic peptide (ANP), troponin I, sarcomeric α-actinin and gap-junction marker connexin-43 in engrafted Muse cells. Confocal microscopy showed that green fluorescent protein (GFP) used to label Muse cells merged with ANP, troponin I and sarcomeric α-actinin around the border area. Expression of cardiac markers on Muse cells confirmed differentiation of these cells into cardiomyocytes (Fig. 4A, 4B and 4C). Moreover, the expression of connexin 43 between the Muse cells that had differentiated into cardiomyocytes and the host cardiomyocytes suggested potential intercellular propagation of excitation and signalling between these cells via gap junctions (Fig. 4D). Two months after AMI, GFP-labelled Muse cells expressed lower levels of ANP and higher levels of troponin I and sarcomeric α-actinin (Fig. 4E, 4F, 4G and 4H). Sarcomeric α-actinin-positive cells showed clear striations characteristic of mature cardiomyocytes (Fig. 4H). GFP-labelled allograft Muse cells also expressed ANP, troponin I and actinin at 2 weeks and still expressed troponin I and actinin at 6 months19. These results indicate that cardiomyocytes that had differentiated from allograft Muse cells remained in the heart for at least 6 months19.
![Figure 4. A–D, GFP-labelled autograft Muse cells engrafted into the infarcted myocardial region expressed cardiac markers ANP, troponin I and α-actinin (2 weeks after AMI). GFP-labelled Muse cells also expressed connexin-43 between themselves and host cardiomyocytes (2 weeks after AMI). E–H, GFP-labelled autograft Muse cells engrafted into the infarct myocardial region expressed cardiac markers ANP, troponin I and α-actinin (2 months after AMI). AMI, acute myocardial infarction; ANP, atrial natriuretic peptide; GFP, green fluorescent protein. [Reproduced from Ref. 19]](../../cms/figure/index/5d8d936376c90031a2262719.jpg)
Figure 4. A–D, GFP-labelled autograft Muse cells engrafted into the infarcted myocardial region expressed cardiac markers ANP, troponin I and α-actinin (2 weeks after AMI). GFP-labelled Muse cells also expressed connexin-43 between themselves and host cardiomyocytes (2 weeks after AMI). E–H, GFP-labelled autograft Muse cells engrafted into the infarct myocardial region expressed cardiac markers ANP, troponin I and α-actinin (2 months after AMI). AMI, acute myocardial infarction; ANP, atrial natriuretic peptide; GFP, green fluorescent protein. [Reproduced from Ref. 19]
From Ref. 19 © 2019 American Heart Association, Inc.
We then investigated whether cardiomyocytes that had differentiated from Muse cells were playing a functional role as working myocardium in the beating heart. GCaMP3-labelled human Muse cells were transplanted into AMI cardiac tissue in the rabbit AMI model. GCaMP is a calcium sensor protein obtained by genetically engineered fusion of enhanced GFP (EGFP), calmodulin (CaM) and myosin light chain fragment (M13). When a calcium ion binds to calmodulin, an interaction between the Ca2+/CaM complex and M13 induces a conformational change in EGFP (fluorophore), which changes the fluorescence intensity. This change in GCaMP fluorescence intensity can be used to detect changes in the calcium concentration. Two weeks after infarction, thoracotomy was performed to observe the postinfarct heart with an epifluorescence stereomicroscope (Nikon, SMZ745T) equipped with an EXFO X-Cite® illumination source. A time-intensity curve showed that a green signal synchronous with ECG recordings was intensified during systole and became less intense during diastole19. This signal represented the changes in intracellular Ca2+ concentration in cardiomyocytes that had differentiated from Muse cells, demonstrating that differentiated cardiomyocytes were functioning as working myocardium.
3.4 Differentiation of Muse cells into blood vessels and paracrine effect
Two weeks after AMI, GFP-labelled Muse cells expressed vascular endothelial marker CD31 and vascular smooth muscle cell marker α-smooth muscle actin, and they formed blood vessels. The Muse group had significantly more capillaries in the border area than the control, non-Muse and MSC groups (Fig. 5A and 5B). Muse cells have a paracrine effect that is also observed in MSCs. Vascular endothelial growth factor (VEGF), hepatocyte growth factor (HGF), matrix metalloproteinase (MMP)-2 and MMP-9 were detected in the culture supernatant of Muse cells19. After intravenous injection of Muse cells, VEGF, HGF, MMP-2 and MMP-9 were expressed in the infarct border area, as was observed with MSCs. The level of VEGF expression was particularly high compared with MSCs and non-Muse (Fig. 5C). VEGF and HGF are angiogenic factors22,23, and HGF exhibits an anti-apoptotic effect23. MMP-2 and MMP-9 have antifibrotic or fibrolytic effects24. Administration of Muse cells reduced the number of terminal deoxynucleotidyl transferase dUTP nick-end labelling (TUNEL)-positive cells in the infarct border area, indicating that Muse cells have an anti-apoptotic effect (Fig. 5D). The area of the Sirius Red-positive region in the infarct area was also reduced (Fig. 5E). These results indicate that Muse cells are very likely associated with the increased number of capillaries in the border area, the reduced area of the fibrotic scar region in the infarct area, and the reduced infarct size.
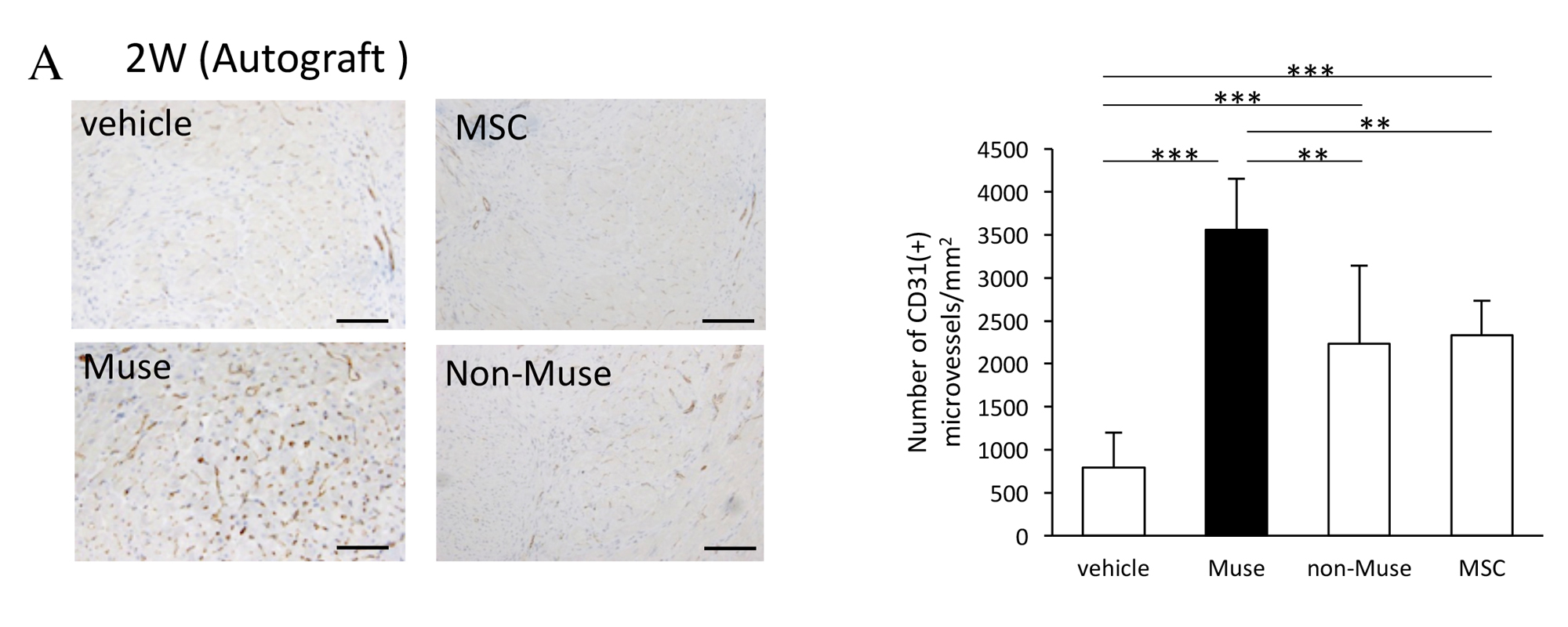
Figure 5. A, Numbers of CD31-positive blood vessels in the infarct border area 2 weeks after AMI (comparison of vehicle control, Muse, non-Muse and MSC groups).
From Ref. 19 © 2019 American Heart Association, Inc.
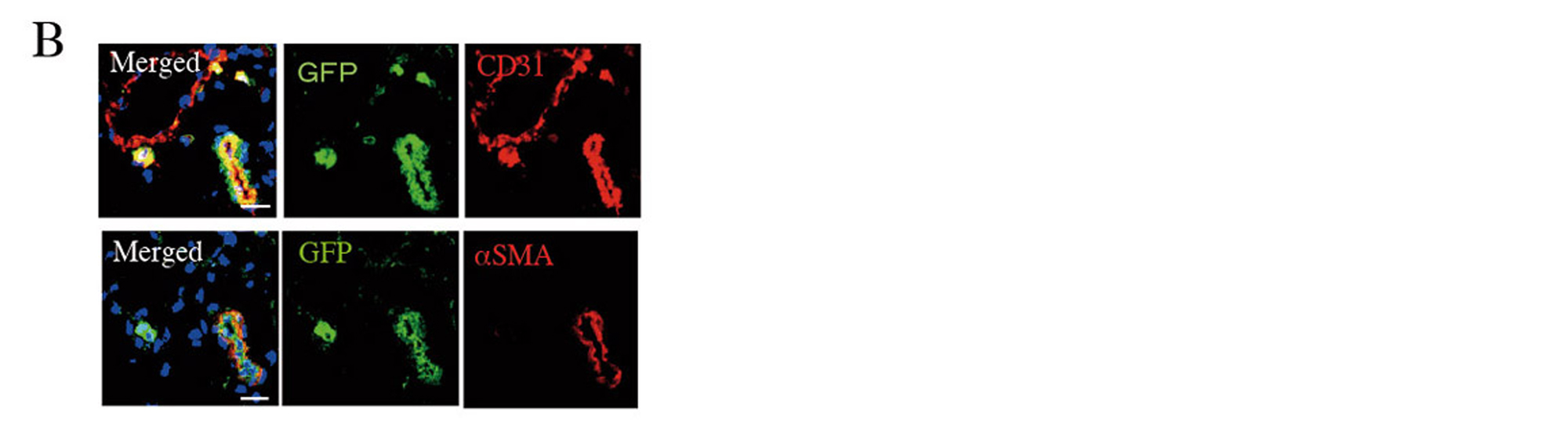
Figure 5. B, GFP-labelled Muse cells expressed vascular endothelial marker CD31 and vascular smooth muscle marker α-smooth muscle actin (2 weeks after AMI).
From Ref. 19 © 2019 American Heart Association, Inc.
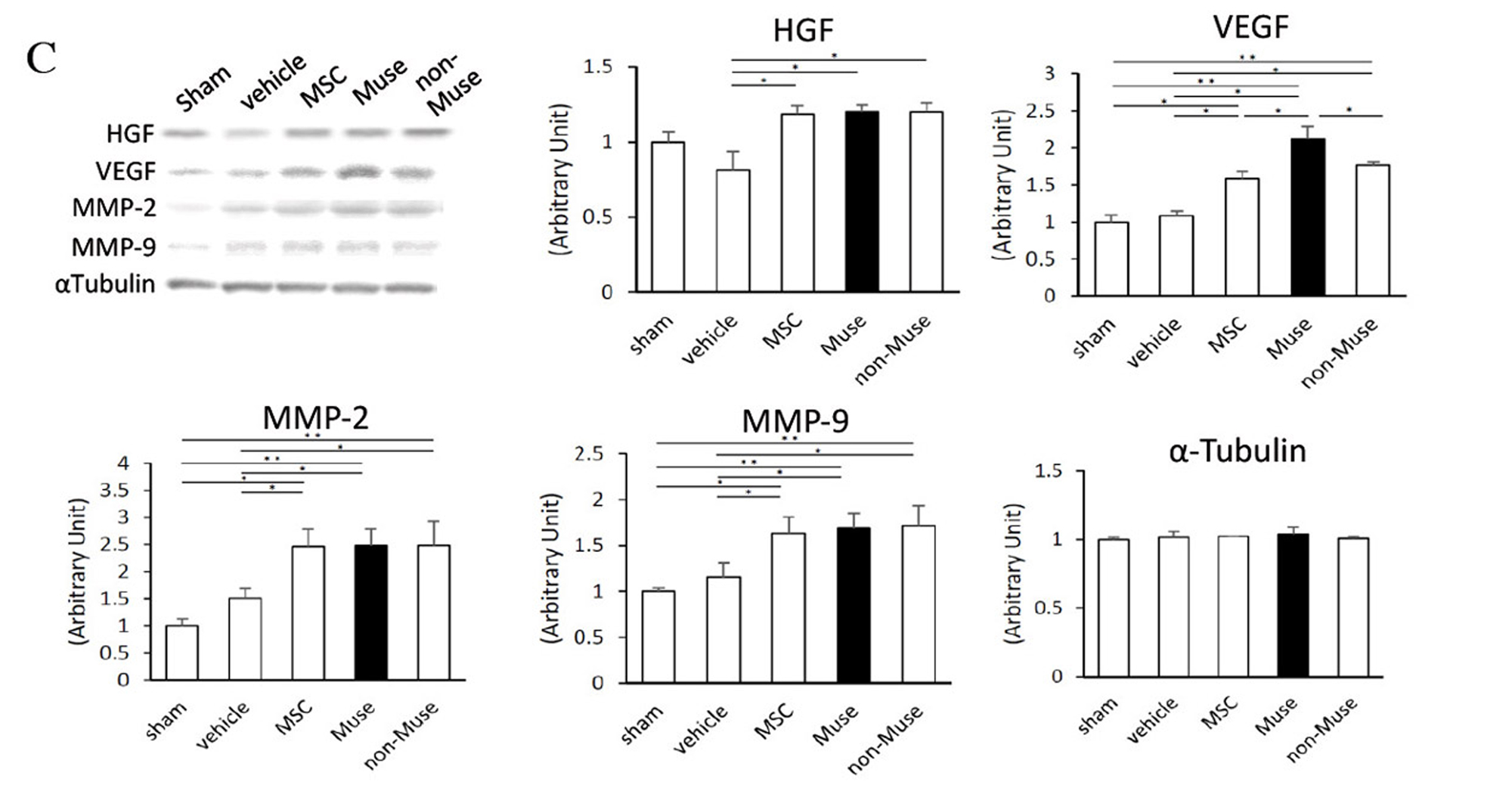
Figure 5. C, Western blot showing the expression of HGF, VEGF, MMP-2 and MMP-9 in the infarct border area 3 days after AMI (vehicle control, Muse, non-Muse and MSC groups).
From Ref. 19 © 2019 American Heart Association, Inc.
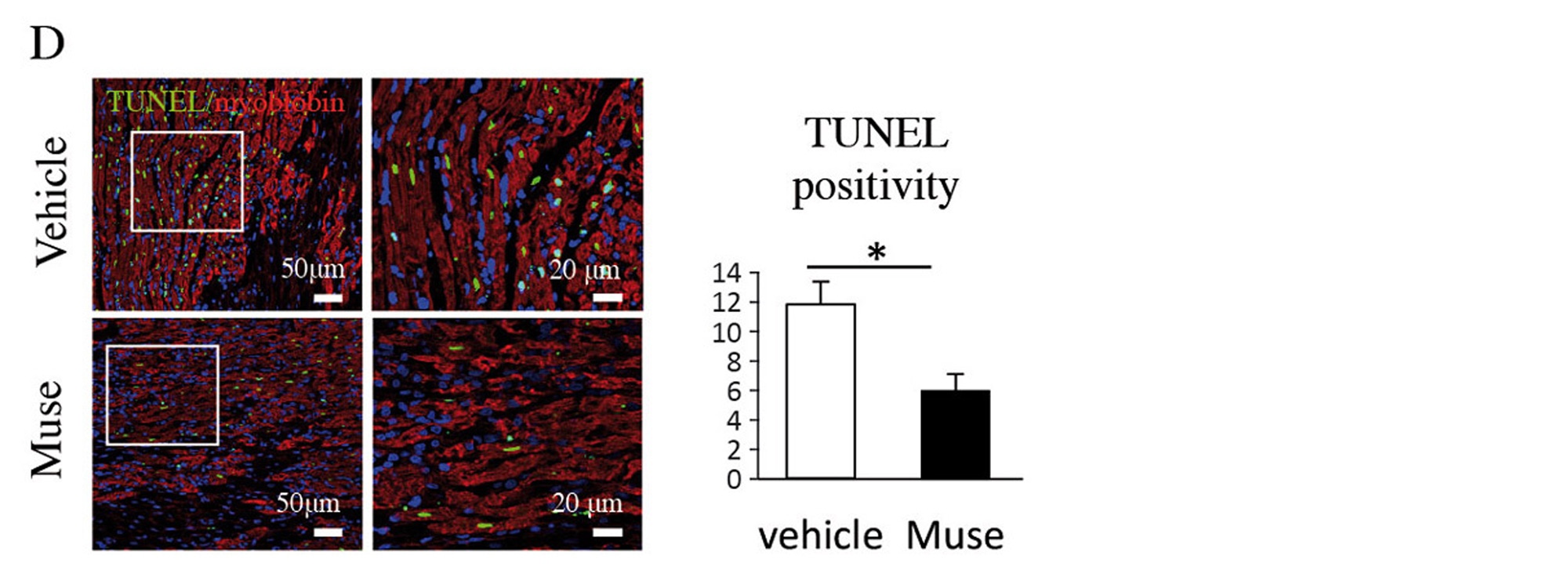
Figure 5. D, Comparison of TUNEL-positive cardiomyocytes in the infarct border area (vehicle and Muse groups).
From Ref. 19 © 2019 American Heart Association, Inc.
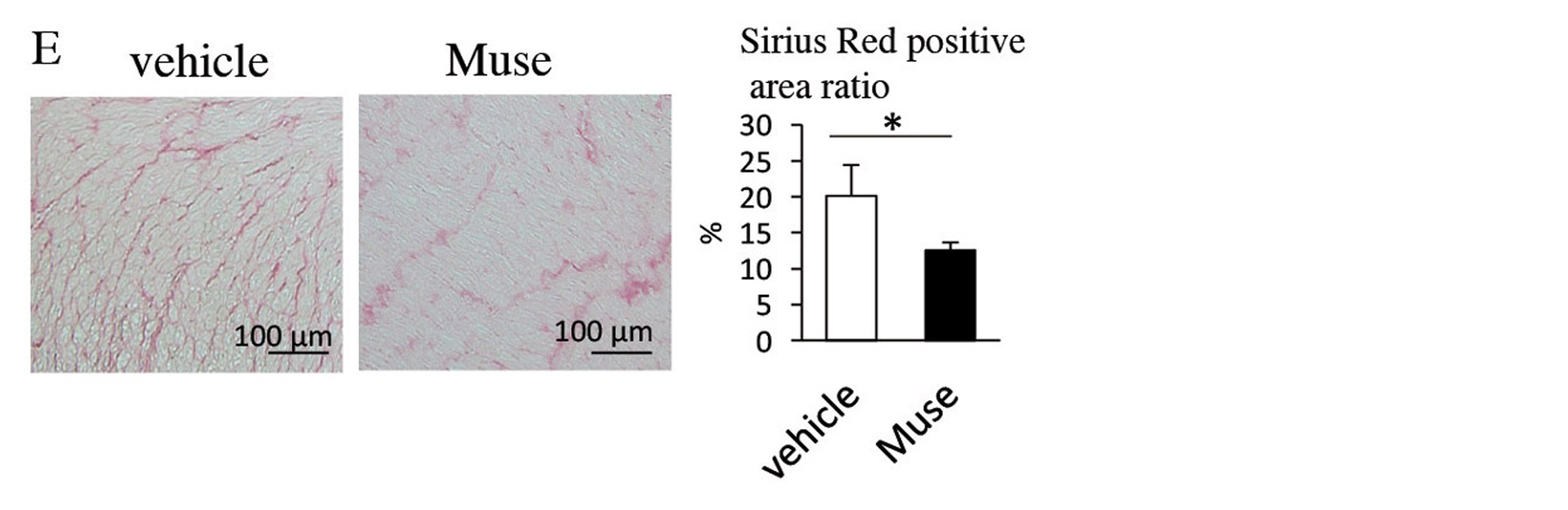
Figure 5. E, Comparison of areas of the Sirius Red-positive region in the infarct regions (vehicle and Muse groups). * p < 0.05; ** p < 0.01; *** p < 0.001; αSMA, α-smooth muscle actin; AMI, acute myocardial infarction; GFP, green fluorescent protein; HGF, hepatocyte growth factor; MMP, matrix metalloproteinase; MSC, mesenchymal stem cell; TUNEL, terminal deoxynucleotidyl transferase dUTP nick-end labelling; VEGF, vascular endothelial growth factor.
From Ref. 19 © 2019 American Heart Association, Inc.
3.5 Immunomodulatory effect of Muse cells
Because Muse cells do not express human leukocyte antigen (HLA)- DR (HLA class II) on the cell surface, they are not attacked by T cells19. Furthermore, because Muse cells express HLA-G, a protein that is present in the placenta to prevent the fetus from being attacked19, they exert immunomodulatory effects and are not attacked by the host receiving Muse cells. These characteristics may partially explain why Muse cells are not eliminated and persist in the infarct area for a long time after engraftment.
3.6 Significance of the persistence of Muse cells in the infarct area
When the rabbit AMI model was co-injected with the selective S1PR2 antagonist JTE-013 and allograft Muse cells, the therapeutic effects of these cells in terms of reducing infarct size and improving cardiac function at 2 weeks were reduced (Fig. 6). When the suicide gene herpes simplex virus thymidine kinase (HSV-tk) was introduced into human Muse cells and these cells were then injected intravenously into AMI rabbits, the human Muse cells were destroyed after engraftment and their effects in terms of reducing infarct size and improving cardiac function (EF) were largely abolished19.
![Figure 6. Effect of sphingosine-1-phosphate receptor 2 (S1PR2) antagonist JTE-013 on the reduction in infarct size and improvement in cardiac function following administration of allograft Muse cells. * p < 0.05; ** p < 0.01; *** p < 0.001. [Reproduced from Ref. 19]](../../cms/figure/index/5dfc6186de2072675042c565.jpg)
Figure 6. Effect of sphingosine-1-phosphate receptor 2 (S1PR2) antagonist JTE-013 on the reduction in infarct size and improvement in cardiac function following administration of allograft Muse cells. * p < 0.05; ** p < 0.01; *** p < 0.001. [Reproduced from Ref. 19]
From Ref. 19 © 2019 American Heart Association, Inc.
Human Muse cells were transplanted after silencing the gene coding for GATA4, a transcription factor expressed during the generation of cardiomyocytes, using siRNA. Reduction in infarct size and EF improvement were significantly attenuated 2 weeks after AMI. Histological analysis showed no differentiation of GFP-labelled Muse cells into cardiomyocytes. The observed attenuation of the effects of the human Muse cells can thus be attributed to blockage of differentiation of the Muse cells into cardiomyocytes by GATA4 silencing19.
These findings indicate that Muse cells are involved in reducing infarct size and improving cardiac function by persisting in the infarct area, differentiating into cardiomyocytes, regenerating blood vessels, and exhibiting paracrine effects such as anti-apoptotic and antifibrotic effects.
References
- Sutton, M. J., Pfeffer, M. A., Moye, L., Plappert, T., Rouleau, J. L. et al. Cardiovascular death and left ventricular remodeling two years after myocardial infarction. Circulation. 96, 3294–3299 (1997). | article
- Reimer, K. A. & Jennings, R. B. The “wavefront phenomenon” of myocardial ischemic cell death. II. Transmural progression of necrosis within the framework of ischemic bed size (myocardium at risk) and collateral flow. Lab. Invest. 40, 633–644 (1979). | article
- Cannon, C. P., Gibson, C. M., Lambrew, C. T., Shoultz, D. A., Levy, D. et al. Relationship of symptom-onset-to balloon time and door-to-balloon time with mortality in patients undergoing angioplasty for acute myocardial infarction. JAMA 283, 2941–2947 (2000). | article
- Moscucci, M. & Eagle, K. A. Door-to-balloon time in primary percutaneous coronary intervention: Is the 90-minute gold standard an unreachable chimera? Circulation. 113, 1048–1050 (2006).| article
- Takemura, G., Ohno, M., Hayakawa, Y., Misao, J., Kanoh, M. et al. Role of apoptosis in the disappearance of infiltrated and proliferated interstitial cells after myocardial infarction. Circ. Res. 82, 1130–1138 (1998). | article
- Minatoguchi, S., Takemura, G., Chen, X.-H., Wang, N., Uno, Y. et al. Acceleration of the healing process and myocardial regeneration may be important as a mechanism of improvement of cardiac function and remodeling by postinfarction granulocyte colony-stimulating factor treatment. Circulation. 109, 2572–2580 (2004). | article
George, J. C. Stem cell therapy in acute myocardial infarction: a review of clinical trials. Trans. Res. 155, 10–19 (2010). | article
- Fisher, S. A., Zhang, H., Doree, C., Mathur, A. & Martin-Rendon, E. Stem cell treatment for acute myocardial infarction. Cochrane Database Syst. Rev. 9, CD006536 (2015). | article
- Kuroda, Y., Kitada, M., Wakao, S., Nishikawa, K., Tanimura, Y. et al. Unique multipotent cells in adult human mesenchymal cell populations. Proc. Natl. Acad. Sci. USA 107, 8639–8643 (2010). | article
- Wakao, S., Kitada, M., Kuroda, Y., Shigemoto, T., Matsuse, D. et al. Multilineage-differentiating stress-enduring (Muse) cells are a primary source of induced pluripotent stem cells in human fibroblasts. Proc. Natl. Acad. Sci. USA 108, 9875–9880 (2011). | article
- Kuroda, Y., Wakao, S., Kitada, M., Murakami, T., Nojima, M. et al. Isolation, culture and evaluation of multilineage-differentiating stress-enduring (Muse) cells. Nature Protoc. 8, 1391–1415 (2013). | article
- Heneidi, S., Simerman, A. A., Keller, E., Singh, P., Li, X. et al. Awakened by cellular stress: Isolation and characterization of a novel population of pluripotent stem cells derived from human adipose tissue. PLoS One 8, e64752 (2013). | article
- Kinoshita, K., Kuno, S., Ishimine, H., Aoi, N., Mineda, K. et al. Therapeutic potential of adipose-derived SSEA-3-positive Muse cells for treating diabetic skin ulcers. Stem Cells Transl. Med. 4, 146–155 (2015). | article
- Wakao, S., Akashi, H., Kushida, Y. & Dezawa, M. Muse cells, newly found non-tumorigenic pluripotent stem cells, reside in human mesenchymal tissues. Pathol. Int. 64, 1–9 (2014). | article
- Hori, E., Hayakawa, Y., Hayashi, T., Hori, S., Okamoto, S. et al. Mobilization of pluripotent multilineage-differentiating stress-enduring cells in ischemic stroke. J. Stroke Cerebrovasc. Dis. 25, 1473–1481 (2016). | article
- Tanaka, T., Nishigaki, K., Minatoguchi, S., Nawa, T., Yamada, Y. et al. Mobilized Muse cells after acute myocardial infarction predict cardiac function and remodeling in the chronic phase. Circ. J. 82, 561–571 (2018). | article
- Dezawa, M. Muse cells provide the pluripotency of mesenchymal stem cells: direct contribution of Muse cells to tissue regeneration. Cell Transplant. 25, 849–861 (2016). | article
- Turer, A. T., Mahaffey, K. W., Gallup, D., Weaver, W. D., Christenson, R. H. et al. Enzyme estimates of infarct size correlate with functional and clinical outcomes in the setting of ST-segment elevation myocardial infarction. Curr. Control Trials Cardiovasc. Med. 6, 12 (2005). | article
- Yamada, Y., Wakao, S., Kushida, Y., Minatoguchi, S., Mikami, A. et al. S1P–S1PR2 axis mediates homing of Muse cells into damaged heart for long-lasting tissue repair and functional recovery after acute myocardial infarction. Circ. Res. 122, 1069–1083 (2018). | article
- Nagaya, N., Fujii, T., Iwase, T., Ohgushi, H., Itoh, T. et al. Intravenous administration of mesenchymal stem cells improves cardiac function in rats with acute myocardial infarction through angiogenesis and myogenesis. Am. J. Physiol. Heart Circ. Physiol. 287, H2670–H2676 (2004). | article
- Freyman, T., Polin, G., Osman, H., Crary, J., Lu, M. et al. A quantitative, randomized study evaluating three methods of mesenchymal stem cell delivery following myocardial infarction. Eur. Heart J. 27, 1114–1122 (2006). | article
- Isner, J. M. & Asahara, T. Angiogenesis and vasculogenesis as therapeutic strategies for postnatal neovascularization. J. Clin. Invest. 103, 1231–1236 (1999). | article
- Chen, X. H., Minatoguchi, S., Kosai, K., Yuge, K., Takahashi, T. et al. In vivo hepatocyte growth factor gene transfer reduces myocardial ischemia-reperfusion injury through its multiple actions. J. Card. Fail. 13, 874–883 (2007). | article
- Mias, C., Lairez, O., Trouche, E., Roncalli, J., Calise, D. et al. Mesenchymal stem cells promote matrix metalloproteinase secretion by cardiac fibroblasts and reduce cardiac ventricular fibrosis after myocardial infarction. Stem Cells 27, 2734–2743 (2009). | article