Author affiliation: Department of Neural Regenerative Medicine, Research Institute for Frontier Medicine, Sapporo Medical University School of Medicine, Sapporo, Japan.
Current treatments for cerebral infarctions and spinal cord injuries are unsatisfactory. Researchers at Sapporo Medical University supported by the Translational Research Center for Medical Innovation (TRI) in Japan have been conducting transplantation experiments using mesenchymal stem cells (MSCs) as donors in animal models of cerebral infarction and spinal cord injury. The results indicate the remarkable therapeutic benefits of intravenous administration of these cells. A clinical study with subacute cerebral infarction patients suggested the safety and potential therapeutic effects of autologous MSCs that had been cultured using autologous human serum and administered by a single intravenous infusion. A clinical trial on the application to cerebral infarction is ongoing, while a clinical trial on the application of MSCs to spinal cord injuries has also been completed. MSCs have the potential to cause a paradigm shift in treatment strategies for refractory neurological diseases.
1. CEREBRAL INFARCTION
1.1 Introduction
Cerebral infarction is a disease that still lacks a cure, and recovery from the residual neurological dysfunction it causes is extremely difficult. Its annual incidence in Japan is just under 300,000. Most patients die or are left with a serious residual disability. An estimated 5.2 million people in Japan will need nursing care by 2025. The number of people with risk factors for cerebral infarction (such as diabetes, hypertension and hyperlipidemia) currently exceeds 10 million, and it is expected to continue rise as Japan’s population ages. The social burden of cerebral infarction is enormous — annual healthcare costs are estimated to be about 2 trillion yen and social costs about 8 trillion yen.
Treatment to date is based on the concept that the therapeutic window after the onset of cerebral infarction is very short. The harsh reality is that no effective treatment currently exists for infarct lesions once they are complete. The gold standard has thus been to detect the ischemia site before an infarct is complete and to provide intensive treatment focused on thrombolytic therapy within a very limited period after onset. Current standard treatment is both medical (including antiplatelet and anticoagulant therapies) and surgical (including cerebral revascularization), but the results are unsatisfactory. Intravenous tissue plasminogen activator therapy, a promising hyperacute treatment, has also been used, but only a limited number of patients are eligible for it, since it must be given within 4.5 hours of onset.
It is thus difficult to treat cerebral infarction. Brain tissue is quickly damaged, and it is difficult to start effective treatment during this time. Furthermore, there is no established treatment for regenerating central nervous tissue that has been damaged by ischemia. Rehabilitation is the only treatment for disability after cerebral infarction.
A new method of treatment is thus needed. With the recent advances in neuroscience and stem-cell research, it is becoming increasingly possible to treat central nervous system disorders using regenerative medicine. However, although many studies have been conducted worldwide, covering many areas of basic research, most have not yet been translated into clinical practice.
Since the early 1990s, we have been actively conducting transplantation experiments using different types of stem cells as donors in animal models of central neurological disease, including cerebral infarction model1-4. From the mid-1990s, we have focused on nerve regeneration research using bone marrow cells, which were expected to be closest to clinical application, as donor cells5–8. In particular, we have focused on mesenchymal stem cells (MSCs) that have a strong regenerative effect on the nervous system and have proven to be a useful source of donor cells. We have reported many basic research results that show the remarkable therapeutic effects of intravenous administration of these cells in animals with various neurological disease models, including cerebral infarction9–27, spinal cord injury28,29 and Parkinson’s disease28,29. Based on the preclinical proof-of-concept studies to date, the mechanisms of action of MSCs may include:
- accumulation of transplanted cells in the lesions (homing effect)10,13,20,30
- neurotrophic, neuroprotective and anti-inflammatory effects of transplanted cells via neurotrophic factors11,14,16,20
- remyelination of demyelinated axons5,7,28,31
- neural regeneration (differentiation into neural cells), regeneration of damaged axons and axonal sprouting28,31
- stabilization of the blood–brain barrier (BBB)17,28,32
Since transplanted cells exert therapeutic effects over time on different processes and at different sites, we can expect good therapeutic effects from a single treatment.
Following on from this basic research, we started a clinical study in January 200733,34 (Fig. 1). We evaluated the safety and therapeutic effects of autologous MSCs that had been cultured and expanded using autologous human serum in patients with subacute cerebral infarction. MSCs were administered by a single intravenous infusion rather than by surgery. We obtained data showing a certain improvement in recovery compared with the course of symptoms seen with conventional treatment. These results were evaluated based on imaging findings and clinical symptoms33,34.
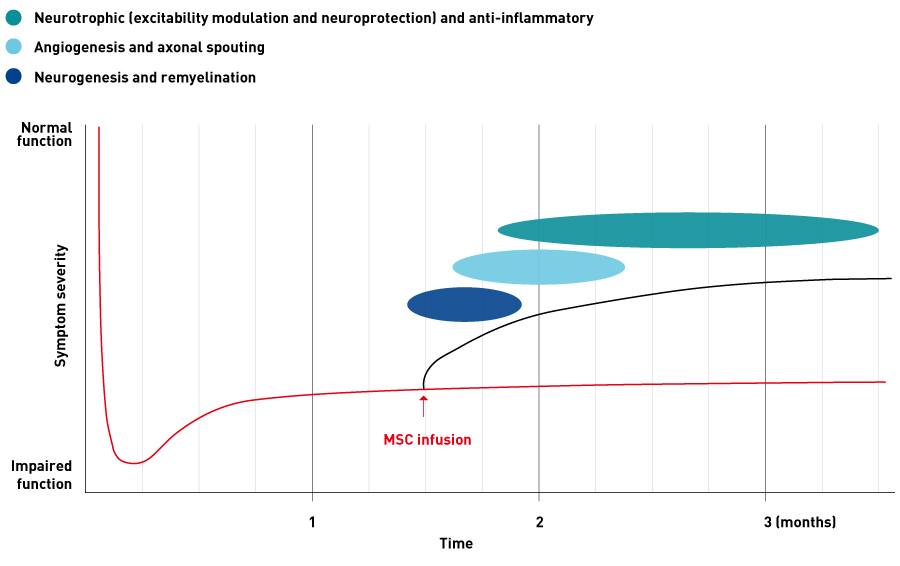
Figure 1. Schematic plot of symptom severity against time following administration of mesenchymal stem cells.
Modified from Ref. 34, copyright (2019), with permission from Elsevier
The potential therapeutic effects produced by this treatment come from minimizing the neural damage caused by cerebral infarction and facilitating recovery (regeneration) of the nervous system. It is difficult to repair the damaged brain using conventional treatment. In contrast, this new treatment is expected to actively repair the ischemic brain via the multifaceted mechanisms described above. The proposed indication for this investigational treatment is improvements in neurological symptoms and signs, in impaired daily activities and in functional disability associated with cerebral infarction.
1.2. Clinical research
1.2.1 Overview
Autologous MSCs were cultured with autologous human serum and intravenously infused into 12 patients with supratentorial cerebral infarction33. Patients were enrolled in the study during the subacute phase after receiving standard treatment for acute cerebral infarction. Causes of cerebral infarction could be any within the NINDS-III classification, but infratentorial infarcts, such as cerebellar infarction and brain stem infarction, were excluded. Mild cases or extremely severe cases were excluded (patients with a modified Rankin scale score of 3–5 and Japan Coma Scale score of 0–100 were included). When all patients had been examined and approved by the Evaluation Committee, bone marrow aspirates (up to 60 ml) were collected from the iliac crest under local anesthesia by a haematologist. MSCs were then cultured in a specialized culture facility (the Cell Processing Center) for 2 to 3 weeks until the target cell number of 1 × 108 cells was reached. The cells were then frozen and their safety and quality tested; only those that passed the tests were administered. Administration was by intravenous infusion into a peripheral vein over 30–60 minutes. The study included nine men and three women, with ages ranging from 41 to 73 years (59.2 ± 8.2 years); 12 patients had motor paralysis and 5 patients had aphasia. The patients received cell transplantation between 36 and 133 days after the onset of cerebral infarction. Assessments included general blood lab tests such as CBC, magnetic resonance imaging (MRI), and clinical symptom evaluation according to the NIH stroke scale (NIHSS) and the modified Rankin scale (mRS).
According to NIHSS and mRS evaluation, the speed of recovery was significantly accelerated by transplantation (Fig. 2)33. Although spontaneous recovery, which occurs as part of the natural course of cerebral infarction, usually slows down during the subacute phase, further acceleration of symptom recovery occurred.
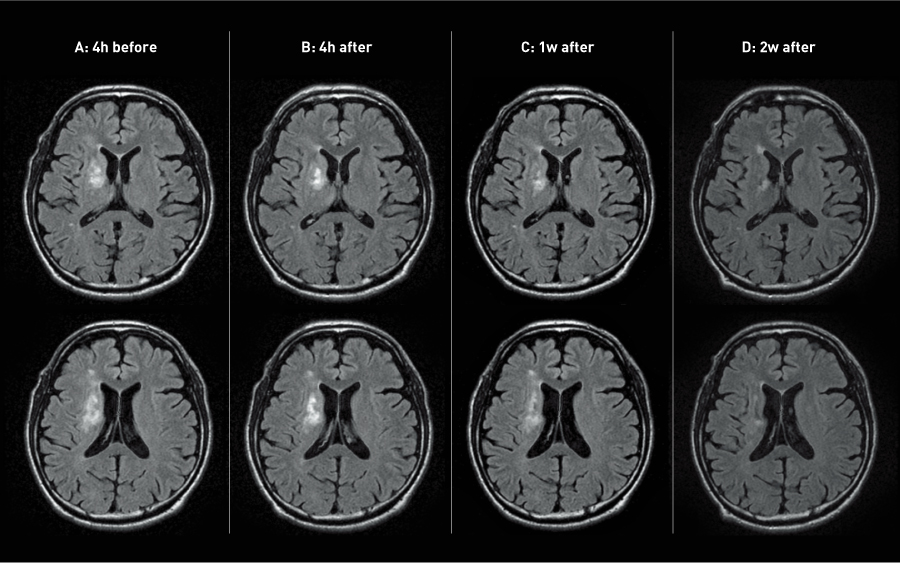
Figure 2. Magnetic resonance imaging images of patients’ brains before and after the administration of mesenchymal stem cells.
© 2019 Oxford University Press
After cerebral infarction, changes in MRI (FLAIR) over time generally converge after approximately 1 month, the high-intensity area being very similar in size, density and shape to the final infarct lesion. However, although transplantation was performed more than 30 days after onset, high-intensity areas on MRI (FLAIR) still showed a statistically significant reduction in size and density after transplantation (Fig. 3)33.
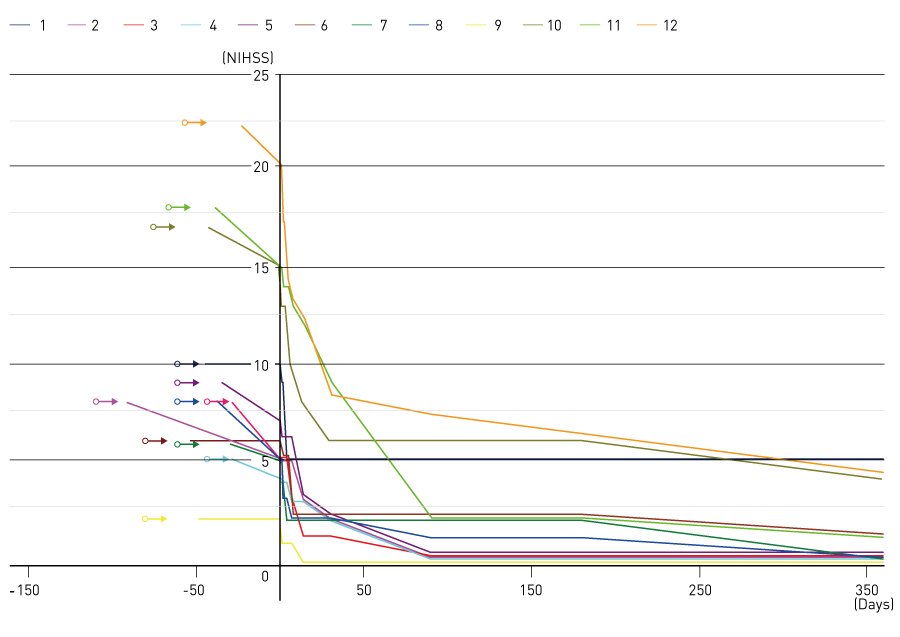
Figure 3. Effect of administration of mesenchymal stem cells on the NIH stroke scale (NIHSS) of patients.
© Modified from Ref. 34, copyright (2019), with permission from Elsevier
1.2.2 What can we learn from these results?
We need to recognize the importance of having multiple points of action when developing new treatments for cerebral infarction. Over the past few decades, many drug candidates have been investigated, but their therapeutic effects are limited. The mechanism of action of a drug may be very clear when it only has limited mechanisms of action. However, the conditions after cerebral infarction vary considerably and are highly complex, as if a storm is raging in the ischemic brain. Why is cell therapy successful in such conditions? We believe the answer lies in the diversity of mechanisms of action of cell therapy. As described above, cell therapy acts in various ways over time on different processes and at different locations, and this sets it apart from current pharmaceutical treatments.
We must reconsider the pathophysiology of cerebral infarction, in particular the conventional wisdom that brain tissue damage progresses irreversibly in a very short period after cerebral infarction, so that is impossible to treat the brain once it has been damaged. The therapeutic time window is thus thought to be limited to the hyperacute phase, and treatment is focused on revascularization and neuroprotection. As described above, cerebral infarction regions treated 6 weeks or more after the onset of cerebral infarction recovered very quickly after cell therapy. This indicates that there are treatable brain tissues remaining in regions where we previously believed that irreversible damage had occurred. This does not refer to the long-term existence of the penumbra; rather, neurons and axons can be dysfunctional but remain anatomically alive for a long time.
We also must treat the entire central nervous system, not just the damaged brain. Many people tend to think of regenerative medicine only as regeneration of damaged brain tissues. However, they are failing to see the forest for the trees. The brain has a larger backup system than we think. In evolutionary history, the nervous system, which is crucial to our survival, encountered many challenges and acquired various backup systems. When trying to reduce disability after cerebral infarction, regenerating the damaged brain is certainly important but so is increasing the plasticity of the healthy areas of the brain. Indeed, increased plasticity is particularly noticeable after cell therapy. In regenerative medicine for cerebral infarction, we must make a paradigm shift towards treating the entire central nervous system.
And we need to recognize the importance of self-healing. Among the various types of stem cells currently available, why does intravenous infusion of high-dose MSCs produce such a potential therapeutic effect? The answer lies in the role that MSCs play under normal circumstances. Even when we are healthy, MSCs seem to be released from the bone marrow into the peripheral circulation and are likely to be involved in maintaining the function of organs and in healing wounds throughout our body. MSCs are thought to be stem cells that make a considerable contribution to self-healing. This sets them apart from other stem cells. Any healthcare intervention, whether drug treatment or surgery, relies on the self-healing that follows. The more we learn about medicine, the less we know, and hence we must focus on maximizing self-healing abilities.
1.3. Treatment mechanism
1.3.1 The blood–brain barrier and the homing effect
It is well known that when central nervous system (CNS) tissues are damaged by ischemia, the BBB is destroyed, and this BBB dysfunction often lasts for about 1 month, depending on the extent of ischemia. To evaluate the extent to which BBB destruction is involved in the accumulation mechanism (homing effect) of intravenous MSCs in cerebral infarction lesions, we used a rat model of permanent middle cerebral artery occlusion. In this cerebral infarction model, BBB breakdown lasted for approximately 2 weeks and was mostly repaired after 4 weeks13. However, intravenously administered MSCs continued to accumulate in the lesions in the same manner more than 4 weeks after ischemic injury13. This suggests that breakdown of the BBB is not needed for MSCs to migrate from the blood vessels to the brain parenchyma. An experiment with a mouse model of prion disease, in which there is minimal BBB destruction, showed a similar homing effect35. We conclude that sufficient numbers of intravenously administered MSCs can reach lesions even in subacute or chronic cerebral infarction where repair of the BBB is almost complete19, and can reach lesions not only in supratentorial but also in infratentorial regions18.
1.3.2 Multilevel mechanisms of action
We have published many basic research findings on the several possible mechanisms involved in using MSCs to treat cerebral infarction. These mechanisms fall into three main groups: neurotrophic and neuroprotective effects by neurotrophic factors11,14,16,20, angiogenic effects (recovery of cerebral blood flow)22,26,27 and neural regeneration10,11,14,15,20,25,28,29,36,37. We presume that these therapeutic effects are produced at different times. Neurotrophic and neuroprotective effects by neurotrophic factors occur very quickly, in a matter of hours, because humoral factors produced by MSCs act directly on the cerebral infarct lesion. Angiogenic effects start to appear approximately 3 days after the onset of cerebral infarction, and the recovery of cerebral blood flow becomes obvious after 1 week. The effects of neural regeneration first become apparent after 1 week and they increase over at least several months. Although these mechanisms are based on inherences drawn from animal studies, the results of our clinical study33,34 suggest similar multilevel effects also occur in humans.
1.3.3 Mechanisms of action of neurotrophic factors
We confirmed a statistically significant change in clinical symptoms and MRI measurements within 1–2 weeks after cell transplantation. Most patients showed reduced motor paralysis and improved resolution of spasticity within 1–3 days after transplantation, and reduction in the high-intensity area in MRI (FLAIR) within 1–2 weeks. These changes, observed soon after transplantation, are thought to be mainly due to the activity of neurotrophic factors secreted by the transplanted cells. After intravenous administration, transplanted cells accumulate in the cerebral infarct lesion and increase local levels of neurotrophic factors (e.g., brain-derived neurotrophic factor [BDNF]20 and glial-derived neurotrophic factor [GDNF]11) within several days14.
The effects of BDNF and GDNF on brain edema are well known, but these two factors are effective only in reducing edema in the acute phase of cerebral infarction. The patients in our clinical study were subacute cases, so this mechanism may differ slightly from the effects on regular brain edema. It may be more accurate to say that the high-intensity area on MRI (FLAIR) during this phase indicates a condition where intra- and extracellular fluid volumes are high. In current clinical practice, this high-intensity region has been considered dead or close to dying, and hence unable to be reduced using existing drugs (such as osmotic diuretics and steroids). If the recovery of neural function in our patients did indeed result from a reduction in brain edema due to the increased concentration of neurotrophic factors at the local lesion site, we may have to fundamentally reconsider the currently accepted pathophysiological concepts of cerebral infarct lesions. The region we have considered to be untreatable in subacute cerebral infarctions is not in fact damaged beyond repair, but consists of brain tissues that can still be treated.
Not only do neurotrophic factors have anti-edematous and apoptosis-reducing effects14, they also affect neuronal excitability by acting directly on ion channels on the surface membrane of both neural cells and axons33. For example, BDNF causes changes in neuronal excitability by altering the potassium channel kinetics and the properties of the resting membrane potential, polarization of the action potential, and after-hyperpolarization, all of which occur extremely quickly. The excitability of the neural cell changes within milliseconds of BDNF reaching a cell. BDNF also directly affects the efficiency of neurotransmission in the synapses. Therapeutic effects seen during the early stages after transplantation may due to the effects of these neurotrophic factors, which may restore the functions of the surviving dysfunctional neural cells and axons.
We also know that BDNF restores the activity of K–Cl transporters in the spinal cord and increases the effects of GABA, thereby reducing spasticity. Indeed, spasticity reduction was seen within several days after transplantation. These results suggest that cell therapy’s site of action is not limited to the local cerebral infarct; we may have to consider that cell therapy improves the overall function of the brain, spinal cord and nerves. From this perspective, systemic administration by intravenous injection is reasonable.
1.3.4 Angiogenesis and stabilization of the BBB
One of the effects of MSCs during treatment is recovery of cerebral blood flow by angiogenesis22,26,27. There are two possible mechanisms for this: MSCs accumulating in the local cerebral infarct induce angiogenesis by secreting angiogenic factors (e.g., vascular endothelial growth factor [VEGF] and angiopoietin); and transplanted MSCs differentiate into vascular endothelial cells and form new blood vessels. Approximately 90% of the endothelial cells of the new vessels are derived from the host, while the other 10% are derived from donor cells. According to the results of our basic research based on animal studies, recovery of cerebral blood flow due to angiogenesis becomes significant approximately 1 week following transplantation27. A clinical study showed improved cerebral blood flow 1 week after transplantation33.
As part of their effect on blood vessels, MSCs have also been found to repair the disrupted BBB. The BBB is impaired regardless of the cause of injury (i.e., ischemia or trauma). BBB injury recovers to some extent after approximately 1 month; however, recent studies have revealed that the BBB is not completely repaired even in the chronic phase, and injury continues to negatively affect the surrounding brain tissues. Intravenously administered MSCs have been demonstrated to repair this longer-term breakdown of the BBB, leading to therapeutic effects17,28,32.
1.3.5 Neural regeneration
It has been suggested that neural regeneration is involved in the therapeutic action of MSCs. There are two possible mechanisms for this: MSCs accumulating in the local cerebral infarct promote endogenous neurogenesis; and transplanted MSCs differentiate into neuronal and glial cells10,11,14,15,20,25,28,29,36,37. Remyelination due to differentiation of MSCs into myelin-forming cells, and stimulation of axonal sprouting and regeneration have also been reported5,7,28,31. Thus, neural regeneration is believed to involve various multilevel regenerative processes.
1.3.6 Regeneration of the entire central nervous system
MSCs are known to induce regeneration at the local site of injury, but they have also been shown to increase the plasticity of the area surrounding the injured site and of the normal brain on the opposite side15,25,37. These effects are enhanced when combined with rehabilitation24. Thus, for brain/spinal cord regeneration, it is important to treat not only the local site of injury but the entire CNS. For this reason, intravenous administration of the cells, which enables them to be delivered to the entire CNS, is preferred over local transplantation.
1.4 Cell culture: Autologous human serum versus fetal bovine serum
Like any other stem cells, MSCs change during culture depending on their collection site and culture method. For example, even if we use the same basic medium, culturing cells using fetal bovine serum (FBS) will produce cells with different biological characteristics from those produce by culturing cells using autologous human serum. Using autologous human serum reduces the potential risk of infection, for example by prions33. The cells are also maintained in a less differentiated state and have more stable gene expression and more rapid proliferation than cells cultured with FBS33.
Gene expression analysis reveals that angiopoietin-like 4, which inhibits apoptosis, is upregulated in MSCs cultured in autologous human serum, resulting in reduced cell death; in contrast, growth arrest-specific protein 1 and anti-proliferative protein 1 are highly expressed in MSCs cultured in FBS, slowing the proliferation rate.
FBS also induces differentiation into mesenchymal lineages. For example, cells cultured in FBS have high expression levels of genes associated with differentiation into osteoblasts (cytokine-receptor-like factor 1, transmembrane glycoprotein NMB), adipocytes (leptin receptor, inhibitor of DNA binding 4, members of the complement system), and chondrocytes (extracellular matrix genes, cytokine receptor–like factor 1, leptin receptor, ectonucleotide pyrophosphatase/phosphodiesterase 2, transforming growth factor-β, SMAD6, OLF1/EBF-associated zinc finger gene)33.
A further problem with the use of FBS is the development of acute and delayed immune reactions caused by xenogeneic protein contamination. When human MSCs cultured in xenogeneic serum (108 cells) are transplanted, approximately 7–30 mg of xenogeneic serum protein is known to enter the host; side effects caused by xenogeneic protein have been reported33.
1.5 Autologous versus allogeneic transplantation
Since it takes about 1 to 2 weeks to culture autologous MSCs, we need to develop ways to treat acute diseases, for example, cell banking. Cultured autologous MSCs can be stored frozen for more than 10 years long periods. Patients with a history of transient ischemic attack or high-risk factors may have their MSCs cultured, expanded and cryopreserved in advance, so that they can be administered immediately after cerebral infarction.
In patients whose MSCs need to be cultured after the onset of infarction, cell therapy may be combined with traditional treatment methods; for example, MSCs can be cultured while the patient is being treated with hypothermia during the acute phase to delay the damage to the brain tissues.
Although allogeneic transplantation would solve the problem of culture time, significant challenges are associated with it, such as immune rejection. In T cell activation — the starting point of immune rejection — cell-surface MHC molecules bind to the T cell receptor/CD3 complex on the T cell surface and adhesion molecules mediate co-stimulatory signals. Since MSCs are positive for both HLA classes I and II and adhesion molecules (e.g., CD40 and CD59), intercellular adhesion molecule (ICAM)-1, ICAM-2, ICAM-3 and vascular adhesion molecule (VCAM)-1, MSCs are highly likely to become antigen-presenting cells. After transplantation, MSCs differentiate in the host’s body, resulting in higher expression of antigen-presenting molecules. Therefore, co-administration of immunosuppressants is mandatory when transplanting allogeneic MSCs. However, given the short- and long-term side effects of immunosuppressants, their use would be very limited. Even when immunosuppressants are used, allogeneic transplantation is less effective than autologous transplantation. Obtaining large numbers of allogeneic MSCs that are safe and high quality also presents methodological problems. Thus, the use of allogeneic MSCs would be limited to diseases for which autologous MSC transplantation cannot be performed. For the time being, regenerative medicine using autologous MSCs will take precedence.
1.6 Mode of administration
In cell therapy, we must account for the particular challenges of administering suspensions of living cells. First, these cells are not always 100% separated to the single-cell level. Second, not all cells are alive, so that clumps of cells, particularly dead cells, could become sources of emboli. There is very little difference in therapeutic effect between intra-arterial and intravenous administration. If MSCs are to be administered intravascularly, we can ensure both safety and effectiveness with intravenous administration. Intrathecal administration has the advantage that it needs relatively few cells, but it leads to a lower rate of cell accumulation at the lesion site, and it is difficult to deliver the cells deep into the brain. This administration mode cannot be used when cerebrospinal fluid (CSF) flow is blocked.
If MSCs are to be transplanted directly into the brain by stereotactic surgery, as has been done in small-animal studies, they would need to be injected at multiple points because of the larger size of the human brain. This would increase the risk of bleeding, infection and additional injury. Furthermore, there are no criteria for determining appropriate transplantation sites.
Although it requires administering a relatively high number of cells, intravenous administration is considered best in terms of safety, cell delivery, targeting, effectiveness, versatility and equipment needed.
1.7 Topics for future investigation
The results of our clinical study raise new questions. Functional recovery in cerebral infarct patients treated with MSC transplantation did not always follow the same course as that observed in patients not treated with MSCs. For example, shortly after MSC transplantation, some patients showed further improvement in volitional control of their peripheral extremities, and some showed improvement in complete flaccid paralysis of their upper extremities. The effects of MSC transplantation are thought to be due to both donor and host cells, and are characterized by various mechanisms operating at different times and different sites. This may explain why the recovery course seen in this study differed from the conventional course. Points to reconsider include the development of new measures of effectiveness, and different approaches to rehabilitation and goal setting.
Most methods of assessing stroke are based on data obtained by observing the course of spontaneous functional recovery. The various recovery processes induced by MSC transplantation, which differ from traditional processes, cannot be fully assessed by traditional methods; we need to develop new measures that account for treatment mechanisms. MSC transplantation is also likely to lead to recovery in smaller motor units, which we need to be able to assess in detail. One possible way is to use objective data obtained from 3D motion analysis. However, we also need a simpler assessment method that does not require special equipment.
Rehabilitation strategies also need to be reconsidered in order to increase the therapeutic effects of MSC transplantation. Neural cells start to regenerate soon after treatment and the process continues for several months; differentiation of donor cells into neural cells and activation of host cells continue, resulting in reconstruction of neural circuits. The key role of rehabilitation is to facilitate this reconstruction effectively and efficiently. We need to investigate what methods are most appropriate for reconstructing neural circuits, making use of existing and new methods. It is important to accurately activate the target neural circuit and increase its use; the key factors are the form, number of sessions and frequency of exercise.
We can expect a change in the overall goal of rehabilitation. Traditionally, the goal has been to help patients return to home and work, based on the prevailing idea that it is difficult to regenerate the impaired brain. In addition to preventing joint contractures and disuse syndrome, rehabilitation aims at maximizing the use of remaining function, using assistive devices to compensate for lost function, and enabling patients to perform daily living activities. Achievement of higher goals such as walking depends on how much function remains. Regenerative medicine using MSC transplantation will overturn current ideas on rehabilitation and will fundamentally change rehabilitation strategies. MSC transplantation promises further improvement in the functions of the extremities and trunk, and so we can set higher goals and see higher success rates.
2. SPINAL CORD INJURY
2.1 The need for a new treatment
Spinal cord injuries occur when the spinal column is subjected to a powerful external force, usually trauma. The most common causes are traffic accidents, followed by sport- or work-related accidents and falls from stairs. There are 100,000 affected patients in Japan, and an estimated 5,000 people are injured each year. The cost of healthcare in the first year after an injury is over 10 million yen per patient; the total annual healthcare cost for those who are newly injured is 50 billion yen. According to a closed commercial report by Thomson Reuters, social security for spinal-cord-injury patients costs more than 10 billion yen globally each year. In addition, there are large financial losses, including lost employment opportunities for patients with spinal cord injury and for family members who provide care.
When the spinal cord is injured, the patient loses movement and sensation in the arms and legs below the level of injury. The higher the injury, the more extensive the paralysis and the more severe the disability. Damage at the thoracic or lumbar level can cause paralysis of the lower body, whereas damage at the cervical level can produce quadriplegia. Unfortunately, it is impossible to regenerate the injured spinal cord using current medical techniques, so development of a new treatment has been eagerly anticipated for years. In Japan, many of these patients are young, and most of them must live with serious disabilities for the rest of their lives.
Currently, there are three standard treatment methods, none of which provide satisfactory outcomes.
1. Decompression and stabilization surgery during the acute phase
It is impossible to repair the injured spinal cord itself by surgery. The main purpose of surgery is to reduce and stabilize fractures and dislocations of the spinal column. A direct therapeutic effect on residual disability cannot be expected from surgery.
2. High-dose methylprednisolone therapy during the acute phase
Within 8 hours of injury, methylprednisolone 30 mg/kg is given over 15 minutes, followed after a further 45 minutes by continuous infusion of 5.4 mg/kg/h for 23 hours. However, this treatment has many side effects, and its therapeutic effect is being re-examined.
3. Rehabilitation
Rehabilitation centres mainly on physical and occupational therapy, in which patients practice activities of daily living with the aim of returning to their social activities. However, rehabilitation has very limited therapeutic effect. Rehabilitation for spinal cord injuries does not aim to recover lost functions; rather its main aim is to make the most effective use of remaining functions and to enable patients to perform activities of daily living.
2.2 Research overview
The basic research we have conducted over many years leads us to expect that autologous MSCs (STR01) (the study drug used in investigator-sponsored trials for cerebral infarction) will also be effective for spinal cord injury28,29,38. We are therefore attempting to expand the indication of this investigational drug to spinal cord injury. We submitted a clinical trial notification in October 2013 and started an investigator-sponsored trial (phase II: open label, exploratory trial). The proposed indication is “improvement of neurological symptoms and signs and functional disability associated with spinal cord injury (therapeutic classification code: other biological products 639).” This trial is registered in the Clinical Trials Registry of the Japan Medical Association Center for Clinical Trials (https://dbcentre3.jmacct.med.or.jp/jmactr/App/JMACTRE02_04/ JMACTRE02_04.aspx?kbn=3&seqno=3923).
Neuroradiological, histological and behavioural improvement has been seen in rat models of spinal cord injury after intravenous transplantation of MSCs28,29,38. Treatment mechanisms may include neuroprotective, neuroregenerative, angiogenic and anti-inflammatory effects28,29,38. Treatment was found to be effective not only in the hyperacute phase29,38 but also in the chronic phase28, demonstrating a relatively broad therapeutic time window.
Most studies on regenerative medicine for spinal cord injury adopt the method of directly transplanting cells into the injury site. However, this necessitates inserting a needle into the injured spinal cord to infuse cells, which risks causing additional damage. Direct transplantation also has limited potential treatment mechanisms. Furthermore, even if efficacy can be demonstrated in animal models, the potential risk is considered too high for humans. In this trial, however, autologous MSCs are delivered through intravenous infusion; this procedure is simpler, less invasive and allows the administered cells to reach the entire damaged area due to their natural capacity to accumulate at the injury site. Treatment is expected to produce excellent results.
Intravenously administered MSCs are known to induce regeneration of the motor areas of the cerebral cortex in rats with spinal cord injury38. To maximize the possible therapeutic effect, it is important to induce regeneration of the entire CNS and not just at the spinal cord injury site. Consequently, intravenous injection, which can deliver cells to the entire CNS, is considered a better administration route.
At present, techniques exists to alleviate the damage of spinal cord injury (surgery and drug therapy during the acute phase, followed by rehabilitation), but no techniques can repair the damaged spinal cord itself. MSC administration could become a breakthrough therapy.
2.2.1 An investigator-sponsored trial of STR01 in spinal cord injury
We aim to establish a completely new treatment for spinal cord injury in this investigator-sponsored trial. The indication for this investigational treatment is “improvement of neurological symptoms and signs, impaired activities of daily living, and functional disability associated with spinal cord injury.”
Basic research and preclinical proof of concept studies conducted to date suggest that the action mechanisms of this treatment may include accumulation of transplanted cells in the lesions (homing effect); neurotrophic, neuroprotective and anti-inflammatory effects of transplanted cells via neurotrophic factors; remyelination of demyelinated axons; neural regeneration (differentiation into neural cells), regeneration of damaged axons and axonal sprouting; stabilization of the blood–spinal cord barrier. Since transplanted cells exert multiple therapeutic effects at different times and sites, we can expect very good therapeutic effects after a single treatment.
This treatment is expected to produce greater therapeutic effects than existing treatments since it will minimize neural damage caused by spinal cord injury, inhibit progression of delayed secondary neural injury and facilitate recovery and regeneration of neural cells and axons. Standard treatment cannot repair the damaged spinal cord. In contrast, this new treatment is expected to actively repair the injured spinal cord by the multifaceted mechanisms described above.
The three measures used to assess improvement of neurological symptoms and signs, impaired activities of daily living and functional disability associated with spinal cord injury are:
- ASIA Impairment Scale, developed by the American Spinal Injury Association (ASIA), which is used as the primary outcome measure for assessing improvement in impaired movement and perception
- International Standards for Neurological and Functional Classification of Spinal Cord Injury (ISCSCI-92) designed by ASIA
- Spinal Cord Independence Measure (SCIM-III), an activity of daily living measure designed specifically for spinal cord injury.
Patients included in the trial are those with cervical cord injury (severe cases categorized as grade A, B or C in the ASIA Classification). First registration occurs within a few weeks of spinal cord injury. This is immediately followed by collection of bone marrow and culture of mesenchymal stem cells. The cells are administered intravenously on day 40, and efficacy and safety are evaluated on day 220 (about 6 months after treatment). This clinical trial has already been completed, and the Japan Ministry of Health, Labor and Welfare officially approved “Conditional/Time-limited Authorization” (28 December 2018).
3. FUTURE PROSPECTS
Intravenous MSC therapy is known to be effective not only for localized brain ischemias such as cerebral infarctions, but also for global cerebral ischemias such as post-resuscitation encephalopathy39. It has thus been suggested that intravenous administration of autologous MSCs will be effective for any type of ischemia and will have a wide range of applications. In short, this treatment can act as a potential neuroprotective agent during ischemia of the brain, spinal cord and nerves, and has various potential uses.
The treatment mechanisms of MSCs are wide ranging and include neurotrophic and neuroprotective effects via neurotrophic factors; angiogenic effects (recovery of blood flow); stabilization of the BBB; neural regeneration; and an increase in plasticity, which is believed to contribute greatly to therapeutic effects during the chronic phase. These effects are seen in both adult24,25 and developing brains37. Intravenous administration of MSCs to a neonatal rat model of cerebral infarction has demonstrated increased plasticity of the normal side of the brain, increased brain volume and number of neural cells, and behavioural improvements37.
MSCs have an inhibitory effect on abnormal neural circuits40. Intravenous administration of MSCs in a rat epilepsy model has reduced epileptic seizures by inhibiting abnormal neural circuits and increasing the number of inhibitory GABA neurons40. Thus, MSCs have complementary effects on the CNS when there is a deficit in neuronal activity, and inhibitory effects when there excessive neuronal activity.
We can therefore safely say that MSCs greatly contribute to self-healing. In animal studies, their effectiveness has already been demonstrated in various neurological diseases including:
- chronic cerebral infarction13,19
- cerebral hemorrhage17
- chronic spinal cord injury28
- post-resuscitation encephalopathy39
- Parkinson’s disease41
- prion disease35
- hypoxic ischemic encephalopathy in the developing brain37
- epilepsy40
- brain tumours42
- peripheral nerve injury43,44
Regenerative medicine using somatic stem cells, including bone marrow transplantation, is expected to be put to practical use very soon because it has a high safety profile, allows for autotransplantation, and some of its techniques are already being used. In particular, since MSCs can be cultured and expanded from very small amounts of bone marrow, they are increasingly being recognized as convenient cells for clinical application.
MSCs have the potential to cause a paradigm shift in treatment strategies for refractory neurological diseases including stroke and spinal cord injury, and we anticipate that the indications for “autologous mesenchymal stem cells (STR01)” to be expanded to many refractory neurological diseases.
References
- Akiyama, Y., Honmou, O., Kato, T., Uede, T., Hashi, K. & Kocsis, J. D. Transplantation of clonal neural precursor cells derived from adult human brain establishes functional peripheral myelin in the rat spinal cord. Exp. Neurol. 167, 27–39 (2001). | article
- Honmou, O., Felts, P. A., Waxman, S. G. & Kocsis, J. D. Restoration of normal conduction properties in demyelinated spinal cord axons in the adult rat by transplantation of exogenous Schwann cells. J. Neurosci. 16, 3199–3208 (1996). | article
- Kato, T., Honmou, O., Uede, T., Hashi, K. & Kocsis, J. D. Transplantation of human olfactory ensheathing cells elicits remyelination of demyelinated rat spinal cord. Glia 30, 209–218 (2000). | article
- Oka S., Honmou O., Akiyama Y., Sasaki M., Houkin K. et al. Autologous transplantation of expanded neural precursor cells into the demyelinated monkey spinal cord. Brain Res. 1030, 94–102 (2004). | article
- Akiyama, Y., Radtke, C., Honmou, O. & Kocsis, J. D. Remyelination of the spinal cord following intravenous delivery of bone marrow cells. Glia 39, 229–236 (2002). | article
- Iihoshi, S., Honmou, O., Houkin, K., Hashi, K. & Kocsis, J. D. A therapeutic window for intravenous administration of autologous bone marrow after cerebral ischemia in adult rats. Brain Res. 1007, 1–9 (2004). | article
- Inoue, M., Honmou, O., Oka, S., Houkin, K., Hashi, K. et al. Comparative analysis of remyelinating potential of focal and intravenous administration of autologous bone marrow cells into the rat demyelinated spinal cord. Glia 44, 111–118 (2003). | article
- Sasaki, M., Honmou, O., Akiyama, Y., Uede, T., Hashi, K. et al. Transplantation of an acutely isolated bone marrow fraction repairs demyelinated adult rat spinal cord axons. Glia 35, 26–34 (2001). | article
- Harada, K., Honmou, O., Liu, H., Bando, M., Houkin, K. et al. Magnetic resonance lactate and lipid signals in rat brain after middle cerebral artery occlusion model. Brain Res. 1134, 206–213 (2007). | article
- Honma, T., Honmou, O., Iihoshi, S., Houkin, K., Hamada, H. et al. Intravenous infusion of immortalized human mesenchymal stem cells protects against injury in a cerebral ischemia model in adult rat. Exp. Neurol. 199, 56–66 (2006). | article
- Horita, Y., Honmou, O., Harada, K., Houkin, K., Hamada, H. et al. Intravenous administration of glial cell line‐derived neurotrophic factor gene-modified human mesenchymal stem cells protects against injury in a cerebral ischemia model in adult rat. J. Neurosci. Res. 84, 1495–1504 (2006). | article
- Kocsis, J. D. & Honmou, O. Bone marrow stem cells in experimental stroke. Prog. Brain Res. 201, 79–98 (2012). | article
- Komatsu, K., Honmou, O., Suzuki, J., Houkin, K., Hamada, H. et al. Therapeutic time window of mesenchymal stem cells derived from bone marrow after cerebral ischemia. Brain Res. 1334, 84–92 (2010). | article
- Liu, H., Honmou, O., Harada, K., Nakamura, K., Houkin, K. et al. Neuroprotection by PlGF gene-modified human mesenchymal stem cells after cerebral ischemia. Brain 129, 2734–2745 (2006). | article
- Nagahama, H., Nakazaki, M., Sasaki, M., Kataoka-Sasaki, Y., Namioka, T. et al. Preservation of interhemispheric cortical connections through corpus callosum following intravenous infusion of mesenchymal stem cells in a rat model of cerebral infarction. Brain Res. 1695, 37–44 (2018). | article
- Nakamura, H., Sasaki, Y., Sasaki, M., Kataoka-Sasaki, Y., Oka, S. et al. Elevated brain derived neurotrophic factor levels in plasma reflect in vivo functional viability of infused mesenchymal stem cells for stroke in rats. J. Neurosurg. Sci. 63, 42–49 (2019). | article
- Nakazaki, M., Sasaki, M., Kataoka-Sasaki, Y., Oka, S., Namioka, T. et al. Intravenous infusion of mesenchymal stem cells inhibits intracranial hemorrhage after recombinant tissue plasminogen activator therapy for transient middle cerebral artery occlusion in rats. J. Neurosurg. 127, 917–926 (2017). | article
- Namioka, A., Namioka, T., Sasaki, M., Kataoka-Sasaki, Y., Oka, S. et al. Intravenous infusion of mesenchymal stem cells for protection against brainstem infarction in a persistent basilar artery occlusion model in the adult rat. J. Neurosurg. https://doi. org/10.3171/2018.4.JNS173121 (2018). | article
- Namioka, T., Namioka, A., Sasaki, M., Kataoka-Sasaki, Y., Oka, S. et al. Intravenous infusion of mesenchymal stem cells promotes functional recovery in a rat model of chronic cerebral infarction. J. Neurosurg. https://doi.org/10.3171/2018.5.JNS18140 (2018). | article
- Nomura, T., Honmou, O., Harada, H., Houkin, K., Hamada, H. et al. I.v. infusion of brain-derived neurotrophic factor gene-modified human mesenchymal stem cells protects against injury in a cerebral ischemia model in adult rat. Neurosci. 136, 161–169 (2005). | article
- Omori, Y., Honmou, O., Harada, K., Suzuki, J., Houkin K. et al. Optimization of a therapeutic protocol for intravenous injection of human mesenchymal stem cells after cerebral ischemia in adult rats. Brain Res. 1236, 30–38 (2008). | article
- Onda, T., Honmou, O., Harada, K., Houkin, K., Hamada, H. et al. Therapeutic benefits by human mesenchymal stem cells (hMSCs) and Ang-1 gene-modified hMSCs after cerebral ischemia. J. Cereb. Blood Flow Metabol. 28, 329–340 (2007). | article
- Sasaki, M., Honmou, O., Radtke, C. & Kocsis, J. D. Development of a middle cerebral artery occlusion model in the nonhuman primate and a safety study of I.V. infusion of human mesenchymal stem cells. PLoS One 6, e26577 (2011). | article
- Sasaki, Y., Sasaki, M., Kataoka-Sasaki, Y., Nakazaki, M., Nagahama, H. et al. Synergic effects of rehabilitation and intravenous infusion of mesenchymal stem cells after stroke in rats. Phys. Ther. 96, 1791–1798 (2016). | article
- Suzuki, J., Sasaki, M., Harada, K., Bando, M., Kataoka, Y. et al. Bilateral cortical hyperactivity detected by fMRI associates with improved motor function following intravenous infusion of mesenchymal stem cells in a rat stroke model. Brain Res. 1497, 15–22 (2013). | article
- Toyama, K., Honmou, O., Harada, K., Suzuki, J., Houkin, K., H. et al. Therapeutic benefits of angiogenetic gene-modified human mesenchymal stem cells after cerebral ischemia. Exp. Neuro. 216, 47–55 (2009). | article
- Ukai, R., Honmou, O., Harada, K., Houkin, K., Hamada, H. et al. Mesenchymal stem cells derived from peripheral blood protects against ischemia. J. Neurotrauma 24, 508–520 (2007). | article
- Morita, T., Sasaki, M., Kataoka-Sasaki, Y., Nakazaki, M., Nagahama, H. et al. Intravenous infusion of mesenchymal stem cells promotes functional recovery in a model of chronic spinal cord injury. Neurosci. 335, 221–231 (2016). | article
- Osaka, M., Honmou, O., Murakami, T., Nonaka, T., Houkin, K. et al. Intravenous administration of mesenchymal stem cells derived from bone marrow after contusive spinal cord injury improves functional outcome. Brain Res. 1343, 226–235 (2010). | article
- Matsushita, T., Kibayashi, T., Katayama, T., Yamashita, Y., Suzuki, S. et al. Mesenchymal stem cells transmigrate across brain microvascular endothelial cell monolayers through transiently formed inter-endothelial gaps. Neurosci. Lett. 8, 41–45 (2011). | article
- Sasaki, M., Radtke, C., Tan, A. M., Zhao, P., Hamada, H. et al. BDNF-hypersecreting human mesenchymal stem cells promote functional recovery, axonal sprouting, and protection of corticospinal neurons after spinal cord injury. J. Neurosci. 29, 14932–14941 (2009). | article
- Matsushita, T., Lankford, K. L., Arroyo, E. J., Sasaki, M., Neyazi, M. et al. Diffuse and persistent blood–spinal cord barrier disruption after contusive spinal cord injury rapidly recovers following intravenous infusion of bone marrow mesenchymal stem cells. Exp. Neurol. 267, 152–164 (2015). | article
- Honmou, O., Houkin, K., Matsunaga, T., Niitsu, Y., Ishiai, S. et al. Intravenous administration of auto serum-expanded autologous mesenchymal stem cells derived from bone marrow into stroke patients. Brain 134, 1790–1807 (2011). | article
- Honmou, O., Onodera, R., Sasaki, M., Waxman, S. G. & Kocsis, J. D. Mesenchymal stem cells: therapeutic outlook for stroke. Trends Mol. Med. 18, 292–297 (2012). | article
- Song, C. H., Honmou, O., Ohsawa, N., Nakamura, K., Hamada, H. et al. Effect of transplantation of bone marrow-derived mesenchymal stem cells on mice infected with prions. J. Virol. 83, 5918–5927 (2009). | article
- Kim, S., Honmou, O., Kato, K., Nonaka, T., Houkin, K. et al. Neural differentiation potential of peripheral blood- and bone-marrow-derived precursor cells. Brain Res. 1123, 27–33 (2006). | article
- Sakai, T., Sasaki, M., Kataoka-Sasaki, Y., Oka, S., Nakazaki, M. et al. Functional recovery after the systemic administration of mesenchymal stem cells in a rat model of neonatal hypoxia-ischemia. J. Neurosurg. Pediatr. 22, 467–599 (2018). | article
- Oshigiri, T., Sasaki, T., Sasaki, M., Kataoka-Sasaki, Y., Nakazaki, M. et al. Intravenous infusion of mesenchymal stem cells alters motor cortex gene expression in a rat model of acute spinal cord injury. J. Neurotrauma 36, 411–420 (2019). | article
- Zheng, W., Honmou, O., Harada, K., Suzuki, J., Liu, H. et al. Therapeutic benefits of human mesenchymal stem cells derived from bone marrow after global cerebral ischemia. Brain Res. 1310, 8–16 (2009). | article
- Fukumura, S., Sasaki, M., Kataoka-Sasaki, Y., Oka, S. & Nakazaki, M. et al. Intravenous infusion of mesenchymal stem cells reduces epileptogenesis in a rat model of status epilepticus. Epilepsy Res. 141, 56–63 (2018). | article
- Inden, M., Takata, K., Nishimura, K., Kitamura, Y., Ashihara, E. et al. Therapeutic effects of human mesenchymal and hematopoietic stem cells on rotenone-treated Parkinsonian mice. J. Neurosci. Res. 91, 62–72 (2013). | article
- Nakamura, K., Ito, Y., Kawano, Y., Kurozumi, K., Kobune, M. et al. Antitumor effect of genetically engineered mesenchymal stem cells in a rat glioma model. Gene Ther. 11, 1155–1164 (2004). | article
- Matsuda, Y., Sasaki, M., Kataoka-Sasaki, Y., Takayanagi, A. & Kobayashi, K. et al. Intravenous infusion of bone marrow-derived mesenchymal stem cells reduces erectile dysfunction following cavernous nerve injury in rats. Sex. Med. 6, 49–57 (2018). | article
- Takayanagi, A., Sasaki, M., Kataoka-Sasaki, Y., Kobayashi, K., Matsuda, Y. et al. Intravenous preload of mesenchymal stem cells rescues erectile function in a rat model of cavernous nerve injury. J. Sex. Med. 12, 1713–1721 (2015). | article